Great observatories work together to stretch the boundaries of what is possible for each. Data from the Chandra X-ray Observatory were used in tandem with the James Webb Space Telescope, for example, to observe the death of a star as it was consumed by a black hole. JWST’s infrared look at this Tidal Disruption Event (TDE) helped show the structure of stellar debris in the accretion disk of the black hole, while Chandra charted the high-energy processes at play in the cataclysmic event. Or have a look at the image below, combining X-ray and infrared data from these two instruments along with the European Space Agency’s XMM-Newton, the Spitzer Space Telescope and optical data from Hubble and the European Southern Observatory's New Technology Telescope to study a range of targets. Image: Four composite images deliver dazzling views from NASA’s Chandra X-ray Observatory and James Webb Space Telescope of two galaxies, a nebula, and a star cluster. Each image combines Chandra’s X-rays — a...
Finding Life Signs around Icy Moons
Europa Clipper is scheduled to launch on October 10, with arrival at Jupiter in 2030. That will keep subsurface oceans on our minds as we tangle with the problems of analyzing water locked under kilometers of ice. Some moons, of course, help us out. Enceladus spews watery materials into space through cracks in its crust, making flybys through its geysers a possibility for snagging samples. Europa Clipper may find further evidence of the much less dramatic plume activity that has been spotted on Europa. Clipper’s SUrface Dust Analyzer (SUDA) would prove vital in such analysis. If cellular material is found in an ice grain snared from an orbital pass, would we be able to detect it? The answer may be found in laboratory work with a common bacteria that thrives in the waters off Alaska. As explained in new work out of the University of Washington and the Freie Universität Berlin, the bacterium Sphingopyxis alaskensis is made to order for such studies. It is smaller than Escherichia coli...
Free-Floating Planets as Interstellar Targets
Just a few weeks ago I wrote about stellar interactions, taking note of a concept advanced by scientists including Ben Zuckerman and Greg Matloff that such stars would make for easier interstellar travel. After all, if a star in its rotation around the Milky Way closes to within half a light year of the Sun, it’s a more feasible destination than Alpha Centauri. Of course, you have to wait for the star to come around, and that takes time. Zuckerman (UCLA), working with Bradley Hansen, has written about the possibility that close encounters are when a civilization will attempt such voyages. I have a further idea along the lines of motion through the galaxy and its advantages to explorers, and it’s one that may not require tens of thousands of years of waiting. We’d like to get to another star system because we’re interested in the planets there, so what if an interstellar planet nudges into nearby space? I’ll ignore Oort Cloud perturbations and the rest to focus on a ‘rogue’ or...
New Angles on Planet Formation
Planet formation is a fascinating subtopic of the exoplanet hunt, and it may just have produced the first exoplanet detection in data that go back as far back as 1981, though the event in question has never been confirmed as being caused by a planet. I learned this through a paper sent me recently by Jean Schneider (Observatoire de Paris), who along with colleague Danielle Briot wrote about the early days of transit searches in a chapter for the Handbook of Exoplanets (Springer, 2018). I want to dig deeper into that chapter in a later post, but for now, I note that the planet Beta Pictoris b, discovered in 2008 and orbiting an infant star 63 light years from Earth, may have transited in 1981, according to subsequent papers on the matter. The debris disk around the primary has long fascinated astronomers and it has been investigated for the possible presence of comet-like bodies and subjected to direct imaging searches, which revealed Beta Pictoris b and confirmed it in 2009. But the...
Musings on Red Dwarf Planets
I'm going to start in the Kuiper Belt this morning before going further out, because the news that the Belt may extend much further than expected reminds us of the nature of exploration. The New Horizons spacecraft, well beyond Pluto’s orbit and approaching 60 AU from the Sun, is finding more dust than expected. Our theoretical models didn’t see that coming. In fact, the dust produced by collisions between Kuiper belt objects was thought to decline as we approached the Belt’s outer edge. So just where is that outer edge? It had been pegged around 50 AU but now looks more like 80 AU, if not further out, a finding corroborated by the fact that New Horizons scientists have used Earth-based resources like the Subaru Telescope in Hawaii to find numerous KBOs beyond the assumed boundary. Is this a new population of Solar System objects, or are we actually seeing something more mundane, such as radiation pressure pushing inner belt dust further out than would be expected? It takes patient...
Otto Struve: A Prescient Look at Exoplanet Detection
Some things just run in families. If you look into the life of Otto Struve, you’ll find that the Russian-born astronomer was the great grandson of Friedrich Georg Wilhelm von Struve, who was himself an astronomer known for his work on binary stars in the 19th Century. Otto’s father was an astronomer as well, as was his grandfather. That’s a lot of familial energy packed into the study of the stars, and the Struve of most recent fame (Otto died in 1963) drew on that energy to produce hundreds of scientific papers. Interestingly, the man who was director at Yerkes and the NRAO observatories was also an early SETI advocate who thought intelligence was rife in the Milky Way. Of Baltic-German descent, Otto Struve might well have become the first person to discover an exoplanet, and therein hangs a tale. Poking around in the history of these matters, I ran into a paper that ran in 1952 in a publication called The Observatory titled “Proposal for a Project of High-Resolution Stellar Radial...
What We Know Now about TRAPPIST-1 (and what we don’t)
Our recent conversations about the likelihood of life elsewhere in the universe emphasize how early in the search we are. Consider recent work on TRAPPIST-1, which draws on JWST data to tell us more about the nature of the seven planets there. On the surface, this seven-planet system around a nearby M-dwarf all but shouts for attention, given that we have three planets in the habitable zone, all of them of terrestrial size, as indeed are all the planets in the system. Moreover, as an ultracool dwarf star, the primary is both tiny and bright in the infrared, just the thing for an instrument like the James Webb Space Telescope to harvest solid data on planetary atmospheres. This is a system, in other words, ripe for atmospheric and perhaps astrobiological investigation, and Michaël Gillon (University of Liége), the key player in discovering its complexities, points in a new paper to how much we’ve already learned. If its star is ultracool, the planetary system at TRAPPIST-1 can also be...
Forbidden Worlds? Theory Clashes with Observation
Back before we knew for sure there were planets around other stars, the universe seemed likely to be ordered. If planet formation was common, then we'd see systems more or less like our own, with rocky inner worlds and gas giants in outer orbits. And if planet formation was a fluke, we'd find few planets to study. All that has, of course, been turned on its head by the abundant discoveries of exoplanets galore. And our Solar System turns out to be anything but a model for the rest of the galaxy. In today's essay, Don Wilkins looks at several recent discoveries that challenge planet formation theory. We can bet that the more we probe the Milky Way, the more we'll find anomalies that challenge our preconceptions. by Don Wilkins The past few decades have not been easy on planet formation theories. Concepts formed on the antiquated Copernican speculation, the commonality of star systems identical to the Solar System, have given way to the strangeness and variety uncovered by Kepler,...
A Resonant Sub-Neptune Harvest at HD 110067
The ancient notion of the ‘music of the spheres’ sounds primitive until you learn something about planetary dynamics. Gravity is wondrous and can nudge planets in a given system into orbits that show an obvious mathematical ratio. Two planets in resonance can emerge, for instance, in a 2:1 ratio, where one goes around its star twice in the time it takes the second to orbit it once. Such linkages might seem almost coincidental to the casual observer until the coincidences begin to pile up. In the exoplanet system at HD 110067, for example, resonance flourishes, so much so that we have six planets moving in a ‘resonance chain.’ No coincidence here, just gravity at work, although an actual coincidence is that just when I finished a post highlighting system dynamics in closely packed environments like TRAPPIST-1 as a ‘brake’ on inbound comets, an international team should reveal HD 110067’s resonance chain. It’s a beauty, for all six planets not only move in harmonic rhythm but also turn...
Tightening Proxima Centauri’s Orbit (and an Intriguing Speculation)
Although I think most astronomers have assumed Proxima Centauri was bound to the central binary at Alpha Centauri, the case wasn’t definitively made until fairly recently. Here we turn to Pierre Kervella (Observatoire de Paris), Frédéric Thévenin (Côte d’Azur Observatory) and Christophe Lovis (Observatoire Astronomique de l'Université de Genève). We last saw Dr. Kervella with reference to a paper on aerographite as a sail material, but his work has appeared frequently in these pages, analyzing mission trajectories and studying the Alpha Centauri system. Here he and his colleagues use HARPS spectrographic data to demonstrate that we have at Centauri a single gravitationally bound triple system. This is important stuff; let me quote the paper on this work to explain why (italics mine): Although statistical considerations are usually invoked to justify that Proxima is probably in a bound state, solid proof from dynamical arguments using astrometric and radial velocity (RV) measurements...
The Odds on Alpha Centauri
How extraordinary that the nearest star to Earth is actually a triple system, the tight central binary visually merged as one bright object, the third star lost in the background field but still a relatively close 13000 or so AU from the others. Humans couldn’t have a better inducement to achieve interstellar flight on the grounds of these stars alone. We get three stellar types: The G-class Centauri A, the K-class Centauri B, both of which are capable of hosting planets, perhaps habitable, of their own. And then we have Proxima Centauri, opening up M-class red dwarf stars to close investigation, and we already know of a planet in the habitable zone there, adding to the zest of the venture. If extraterrestrial beings in a system like this would have even more inducement to travel, with another star’s planets perhaps as close to them as our own system’s worlds are to us, we humans are also spurred to undertake a journey, because 4.2 light years is a mere stone’s throw in the overall...
Exoplanet Detection: Nudging Into the Rayleigh Limit
We’re building some remarkably large telescopes these days. Witness the Giant Magellan Telescope now under construction in Chile’s Atacama desert. It’s to be 200 times more powerful than any research telescope currently in use, with 368 square meters of light collection area. It incorporates seven enormous 8.5 meter mirrors. That makes exoplanet work from the Earth’s surface a viable proposition, but look at the size of the light bucket we need to make it work. Three mirrors like that shown below are now in place, and the University of Arizona’s Mirror Lab is building number 6 now. Image: University of Arizona Richard F. Caris Mirror Lab staff members Damon Jackson (left) and Conrad Vogel (right) in the foreground looking up at the back of primary mirror segment five, April 2019. Credit: Damien Jemison; Giant Magellan Telescope - GMTO Corporation. CC BY-NC-ND 4.0. Imaging an exoplanet from the Earth’s surface is complicated by the Rayleigh Limit, which governs the resolution of our...
Atmospheric Types and the Results from K2-18b
The exoplanet K2-18b has been all over the news lately, with provocative headlines suggesting a life detection because of the possible presence of dimethyl sulfide (DMS), a molecule produced by life on our own planet. Is this a 'Hycean' world, covered with oceans under a hydrogen-rich atmosphere? Almost nine times as massive as Earth, K2-18b is certainly noteworthy, but just how likely are these speculations? Centauri Dreams regular Dave Moore has some thoughts on the matter, and as he has done before in deeply researched articles here, he now zeroes in on the evidence and the limitations of the analysis. This is one exoplanet that turns out to be provocative in a number of ways, some of which will move the search for life forward. by Dave Moore 124 light years away in the constellation of Leo lies an undistinguished M3V red dwarf, K2-18. Two planets are known to orbit this star: K2-18c, a 5.6 Earth mass planet orbiting 6 million miles out, and K2-18b, an 8.6 Earth mass planet...
Tidal Lock or Sporadic Rotation? New Questions re Proxima and TRAPPIST-1
Centauri Dreams regular Dave Moore just passed along a paper of considerable interest for those of us intrigued by planetary systems around red dwarf stars. The nearest known exoplanet of roughly Earth’s mass is Proxima Centauri b, adding emphasis to the question of whether planets in an M-dwarf’s habitable zone can indeed support life. From the standpoint of system dynamics, that often comes down to asking whether such a planet is not so close to its star that it will become tidally locked, and whether habitable climates could persist in those conditions. The topic remains controversial. But there are wide variations between M-dwarf scenarios. We might compare what happens at TRAPPIST-1 to the situation around Proxima Centauri. We have an incomplete view of the Proxima system, there being no transits known, and while we have radial velocity evidence of a second and perhaps a third planet there, the situation is far from fully characterized. But TRAPPIST-1’s superb transit...
A Liquid Water Mechanism for Cold M-dwarf Planets
A search for liquid water on a planetary surface may be too confining when it comes to the wide range of possibilities for supporting life. We see that in our own Solar System. Consider the growing interest in icy moons like Europa and Enceladus, where there is no possibility of surface water but a potentially rich environment under a thick layer of ice. Extending these thoughts into the realm of exoplanets reminds us that our calculations about how many life-bearing worlds are out there may be in need of revision. This is the thrust of work by Lujendra Ojha (Rutgers University) and colleagues, as developed in a paper in Nature Communications and presented at the recent Goldschmidt geochemistry conference in Lyon. What Ojha and team point out is that radiogenic heating can maintain liquid water below the surface of planets in M-dwarf systems, and that added into our astrobiological catalog, such worlds, orbiting a population of stars that takes in 75 percent or more of all stars in...
Earth in Formation: The Accretion of Terrestrial Worlds
It would be useful to have a better handle on how and when water appeared on the early Earth. We know that comets and asteroids can bring water from beyond the ‘snowline,’ that zone demarcated by temperatures beyond which volatiles like water, ammonia or carbon dioxide are cold enough to condense into ice grains. For our Solar System, that distance in our era is 5 AU, roughly the orbital distance of Jupiter, although the snowline would have been somewhat closer to the Sun during the period of planet formation. So we have a mechanism to bring ices into the inner Solar System but don’t know just how large a role incoming ices played in Earth’s development. Knowing more about the emergence of volatiles on Earth would help us frame what we see in other stellar systems, as we evaluate whether or not a given planet may be habitable. Usefully, there are ways to study our planet’s formation that can drill down to its accretion from the materials in the original circumstellar disk. A new...
Tightening our Understanding of Circumbinary Worlds
I’m collecting a number of documents on gravitational wave detection and unusual concepts regarding their use by advanced civilizations. It’s going to take a while for me to go through all these, but as I mentioned in the last post, I plan to zero in on the intriguing notion that civilizations with abilities far beyond our own might use gravitational waves rather than the electromagnetic spectrum to serve as the backbone of their communication system. It’s a science fictional concept for sure, though there may be ways it could be imagined for a sufficiently advanced culture. For today, though, let’s look at a new survey that targets highly unusual planets. Binaries Escorted by Orbiting Planets has an acronym I can get into: BEBOP. It awakens the Charlie Parker in me; I can almost smell the smoky air of a mid-20th century jazz club and hear the clinking of glasses above Parker’s stunning alto work. I was thinking about the great sax player because I had just watched, for about the...
The Prevalence of ‘Jupiters’ around Larger Stars
Work on the Centauri Dreams internals continues, with the unwelcome result that the site has been popped offline twice because of a possible security problem. Needless to say, this has to be resolved before I can move forward on other aspects of the rebuild. While I deal with that issue, let me respond to a few backchannel questions about yesterday’s post on gas giants in red dwarf planetary systems. What I’m being asked about is my comment that gas giants like Jupiter, at similar distances and installation, around other classes of stars are common compared to what we see at red dwarfs. This has been a problematic issue, and the matter is a long way from achieving a consensus among researchers. A moment’s reflection yields the reason: Finding gas giants in outer system orbits around a star like the Sun is no easy matter. Radial velocity is most sensitive when dealing with large planets in tight orbits, which is why the first detections in main sequence stellar systems, beginning back...
A Scarcity of ‘Jupiters’ in Red Dwarf Systems
Gas giant worlds like Jupiter may be uncommon around red dwarf stars, as a number of recent studies have found. It would be useful to tighten up the data, however, because many of the papers on this matter have used stellar samples at the high end of the mass range of M-dwarfs. At the Center for Astrophysics | Harvard & Smithsonian (CfA), Emily Pass and colleagues have gone to work on the question by looking at lower-mass M-dwarfs and working with a lot of them, some 200 in their sample, all within 15 parsecs. The question is not purely academic, for some scientists suggest that the presence of a Jupiter-class planet – not uncommon around G-class stars like the Sun – is a factor in the development of life. Migrating inward from a formation in the first few hundred million years of the Solar System’s existence, Jupiter would have stirred up plenty of icy cometary bodies through gravitational interactions. Impacts from this infall into the inner system likely delivered a great deal...
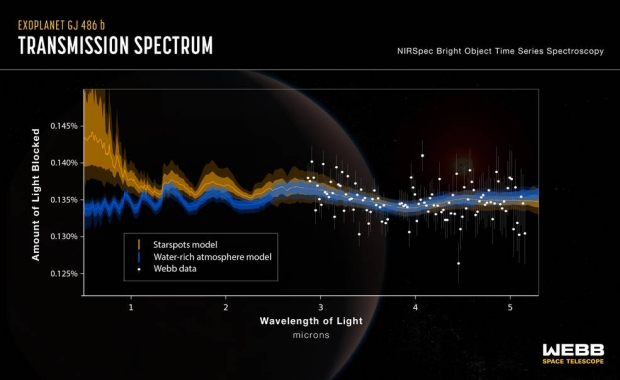
GJ 486b: An Atmosphere around a Rocky M-dwarf Planet?
I might have mentioned the issues involving the James Webb Space Telescope’s MIRI instrument in my earlier post on in-flight maintenance and repair. MIRI is the Mid-Infrared Instrument that last summer had issues with friction in one of the wheels that selects between short, medium and longer wavelengths. Now there seems to be a problem, however slight, that affects the amount of light registered by MIRI’s sensors. The problems seem minor and are under investigation, which is a good thing because we need MIRI’s capabilities to study systems like GJ 486, where a transiting rocky exoplanet may or may not be showing traces of water in an atmosphere that may or may not be there. MIRI should help sort out the issue, which was raised through observations with another JWST instrument, the Near-Infrared Spectrograph (NIRSpec). The latter shows tantalizing evidence of water vapor, but the problem is untangling whether that signal is coming from the rocky planet or the star. This points to an...