If you had to choose, which planetary system would you gauge most likely to house a life-bearing planet: Proxima Centauri or TRAPPIST-1? The question is a bit loaded given that there are seven TRAPPIST-1 planets, hence a much higher chance for success there than in a system that (so far) has produced evidence for only two worlds. But there are other factors having to do with the delivery of prebiotic materials by comet, which is the subject of a new paper from Richard Anslow (Cambridge Institute of Astronomy). “It’s possible that the molecules that led to life on Earth came from comets,’’ Anslow reminds us, “so the same could be true for planets elsewhere in the galaxy.” So let’s untangle this a bit. We don’t know whether comets are vital to the origin of life on Earth or any other world, and Anslow (working with Cambridge colleagues Amy Bonsor and Paul B. Rimmer) does not argue that they are. What their paper does is to examine the environments most likely to be affected by cometary...
Eavesdropping on the Neighbors
Given that interstellar communications have been on my mind recently, I was delighted to receive this essay from Don Wilkins. Based in St. Louis, where he is a now-retired aerospace engineer, Don has plenty of experience in avionics and has the chops to know how to make widely-dispersed aircraft talk to each other. Here his scope is a bit wider: What are the implications of 'lurker' probes, the conceivably ancient (or newer) technologies from an extraterrestrial civilization that might be monitoring our planet? If such exist, their communications become a SETI target, and the question of how their network might operate is an intriguing one. I had no idea, for example, that the idea of gravitational lensing for such communications had made its way into the SETI field, but Don here acquaints us with several studies that tackle the concept, along with other insights as found below. by Don Wilkins If an expansionist star faring civilization exists, it is likely to construct an...
The Order of Interstellar Arrival
Writers have modeled the arrival of an extraterrestrial probe in our Solar System in a number of interesting science fiction texts, from Clarke’s Rendezvous with Rama (1973) to the enigmatic visitors of Ted Chiang’s "Story of Your Life,” which Hollywood translated into the film Arrival (2016). In between I might add the classic ‘saucer landing on the White House lawn’ trope of The Day the Earth Stood Still (1951), based on a Harry Bates short story. All these and many other stories raise the question: What if before we make a radio or optical SETI detection, an extraterrestrial scout actually shows up? Graeme Smith (UC: Santa Cruz) goes to work on the idea in a recent paper in the International Journal of Astrobiology, where he focuses on the mechanism of interstellar dispersion. The model has obvious ramifications for ourselves. We are beings who have begun probing nearby space with vehicles like Pioneer and Voyager, and in our early stages of exploration we could conceivably be...
Galactic Civilizations: Does N=1?
I don’t suppose that Frank Drake intended his famous Drake Equation to be anything more than a pedagogical device, or rather, an illustrative tool to explain what he viewed as the most significant things we would need to know to figure out how many other civilizations might be out there in the galaxy. This was back in 1961, and naturally the equation was all about probabilities, because we didn’t have hard information on most of the factors in the equation. Drake was already searching for radio signals at Green Bank, in the process inventing SETI as practiced through radio telescopes. The factors here should look familiar to most Centauri Dreams readers, but let’s run through them, because among the old hands here we also get an encouraging number of students and people new to the field. N is the number of civilizations with communications potential in the galaxy, with R* the rate of star formation, fp the fraction of stars with planets, ne the number of planets that can support life...
SETI: A New Kind of Stellar Engine
The problem of perspective haunts SETI, and in particular that branch of SETI that has been labeled Dysonian. This discipline, based on Freeman Dyson’s original notion of spheres of power-gathering technology enclosing a star, has given rise to the ongoing search for artifacts in our astronomical data. The fuss over KIC 8462852 (Boyajian’s Star) a few years back involved the possibility that it was orbited by a megastructure of some kind, and thus a demonstration of advanced technology. Jason Wright and team at Penn State have led searches, covered in these pages, for evidence of Dyson spheres in other galaxies. The Dysonian search continues to widen. I cite a problem of perspective in that we have no real notion of what we might find if we finally locate signs of extraterrestrial builders in our data. It’s so comfortable to be a carbon-based biped, but the entities we’re trying to locate may have other ways of evolving. Clément Vidal, a French philosopher and one of the most...
Nucleic Acid Stability in the Venusian Clouds
How to approach finding life on other worlds will continue to be a challenging issue, but how useful that even as we work out strategies for studying exoplanet atmospheres, we have planets we can actually reach right here in our own Solar System. And if the hunt for life has turned up empty thus far on Mars, we can keep searching there even as we consider the exotic possibility of life in the clouds of Venus. We've looked at Venus Life Finder before in these pages. This series of missions is now known as Morning Star, all designed to probe the clouds for signs of a kind of life that would have to endure the most hellish conditions we can imagine. In today's post, Alex Tolley examines the Morning Star Missions and how they might proceed, depending on the results of that all important first sampling of the atmosphere. by Alex Tolley “To boldly seek life, where no terrestrial life has gone before” The “Morning Star Missions” (formerly Venus Life Finder) group had previously outlined...
SETI: New Tools for Screening Out Radio Interference
Two new techniques for examining interesting SETI signals come into view this morning, one out of Breakthrough Listen work at UC-Berkeley, the other from researchers working with the Five-hundred-meter Aperture Spherical radio Telescope (FAST), the so-called ‘Heaven’s Eye’ instrument located in southwest China. In both cases, the focus is on ways to screen SETI observations from disruptive radio frequency interference (RFI), which can appear at first glance to flag a signal from another star. The Chinese work relies upon FAST’s array of receiving instruments, each acting as a separate ‘beam’ to cover slightly different portions of the sky. FAST’s currently operational L-band receiver array consists of 19 beams, to which researchers led by Bo-lun Huang (Beijing Normal University) apply a technique called MultiBeam Point-source Scanning (MBPS). Here the instrument scans the target star sequentially with different beams of the instrument, setting up the possibility of cross-verification...
The “Habitability” of Worlds (Part II)
If we ever thought it would be easy to tell whether a planet was 'habitable' or not, Stephen Dole quickly put the idea to rest when he considered all the factors involved in his study Habitable Planets for Man (1964). In this second part of his essay on habitability, Dave Moore returns to Dole's work and weighs these factors in light of our present knowledge. What I particularly appreciate about this essay in addition to Dave's numerous insights is the fact that he has brought Dole's work back into focus. The original Habitable Planets for Man was a key factor in firing my interest in writing about interstellar issues. And Centauri Dreams reader Mark Olson has just let me know that Dole appears as a major character in a novel by Harry Turtledove called Three Miles Down. It's now in my reading stack. by Dave Moore In Part I of this essay, I listed the requirements for human habitability in Stephen Dole’s report, Habitable Planets for Man. Now I’ll go over what we’ve subsequently...
The “Habitability” of Worlds (Part I)
Dave Moore is a Centauri Dreams regular who has long pursued an interest in the observation and exploration of deep space. He was born and raised in New Zealand, spent time in Australia, and now runs a small business in Klamath Falls, Oregon. He counts Arthur C. Clarke as a childhood hero, and science fiction as an impetus for his acquiring a degree in biology and chemistry. Dave has kept up an active interest in SETI (see If Loud Aliens Explain Human Earliness, Quiet Aliens Are Also Rare) as well as the exoplanet hunt. In the essay below, he examines questions of habitability and how we measure it, issues that resonate in a time when we are preparing to evaluate exoplanets as life-bearing worlds and look for their biosignatures. by Dave Moore In this essay I’ll be examining the meaning of the word ‘habitable’ when applied to planetary bodies. What do we mean when we talk about a habitable planet or a planet’s habitability? What assumptions do we make? The first part of this essay...
Reducing the Search Space with the SETI Ellipsoid
SETI’s task challenges the imagination in every conceivable way, as Don Wilkins points out in the essay below. A retired aerospace engineer with thirty-five years experience in designing, developing, testing, manufacturing and deploying avionics, Don is based in St. Louis, where he is an adjunct instructor of electronics at Washington University. He holds twelve patents and is involved with the university’s efforts at increasing participation in science, technology, engineering, and math. The SETI methodology he explores today offers one way to narrow the observational arena to targets more likely to produce a result. Can spectacular astronomical phenomena serve as a potential marker that could lead us to a technosignature? by Don Wilkins Finite SETI search facilities searching a vast search volume must set priorities for exploration. Dr. Jill Tarter, Chair Emeritus for SETI Research, describes the search space as a “nine-dimensional haystack” composed of three spatial, one temporal...
Abundant Phosphorus in Enceladus Ocean Increases Habitability: But is Enceladus Inhabited?
Finding the right conditions for life off the Earth justifiably drives many a researcher's work, but nailing down just what might make the environment beneath an icy moon's surface benign isn't easy. The recent wave of speculation about Enceladus revolves around the discovery of phosphorus, a key ingredient for the kind of life we are familiar with. But Alex Tolley speculates in the essay below that we really don't have a handle on what this discovery means. There's a long way between 'habitable' and 'inhabited,' and many data points remain to be analyzed, most of which we have yet to collect. Can we gain the knowledge we need from a future Enceladus plume mission? by Alex Tolley There has been abundant speculation about the possibility of life in the subsurface oceans of icy moons. Europa’s oceans with possible hydrothermal vents mimicking Earth’s abyssal oceans and the probable site of the origin of life, caught our attention now that Mars has no extant surface life. Arthur C...
SETI: Asking the Right Questions
Did Carl Sagan play a role in the famous Arecibo message transmitted toward the Hercules Cluster in 1974? I’ve always assumed so, given Sagan’s connection with Frank Drake, who was then at Cornell University, where Sagan spent most of his career. But opinion seems to vary. Artist/scientist Joe Davis, who now has affiliations with both MIT’s Laboratory of Molecular Structure and Harvard Medical School, noted in an email this morning that Sagan’s widow, Ann Druyan, supports the connection, but according to Davis, Drake himself denied Sagan’s role in the composition or transmission of the message. I mention all this because of Tuesday’s post on the simulated SETI signal being sent via ESO’s Mars ExoMars Trace Gas Orbiter, as a kind of work of art in its own right as well as a test case in building public involvement in the decoding of an unusual message. The idea of doing that irresistibly recalled Joe Davis because in 1988 Davis performed his own act of scientific art involving SETI,...
First Contact: A Global Simulation
Now and again scientists think of interesting ways to use our space missions in contexts for which they were not designed. I’m thinking, for example, of the ‘pale blue dot’ image snapped by Voyager 1 in 1990, an iconic view that forcibly speaks to the immensity of the universe and the smallness of the place we inhabit. Voyager’s cameras, we might recall, were added only after a debate among mission designers, some of whom argued that the mission could proceed without any cameras aboard. Fortunately, the camera advocates won, with results we’re all familiar with. Now we have a project out of The SETI Institute that would use a European Space Agency mission in a novel way, one that also challenges our thinking about our place in the cosmos. Daniela de Paulis, who serves as artist in residence at the institute, is working across numerous disciplines with researchers involved in SETI and astronautics to create A Sign in Space, the creation of an ‘extraterrestrial’ message. This is not a...
Assembly theory (AT) – A New Approach to Detecting Extraterrestrial Life Unrecognizable by Present Technologies
With landers on places like Enceladus conceivable in the not distant future, how we might recognize extraterrestrial life if and when we run into it is no small matter. But maybe we can draw conclusions by addressing the complexity of an object, calculating what it would take to produce it. Don Wilkins considers this approach in today's essay as he lays out the background of Assembly Theory. A retired aerospace engineer with thirty-five years experience in designing, developing, testing, manufacturing and deploying avionics, Don tells me he has been an avid supporter of space flight and exploration all the way back to the days of Project Mercury. Based in St. Louis, where he is an adjunct instructor of electronics at Washington University, Don holds twelve patents and is involved with the university’s efforts at increasing participation in science, technology, engineering, and math. Have a look at how we might deploy AT methods not only in our system but around other stars. by Don...
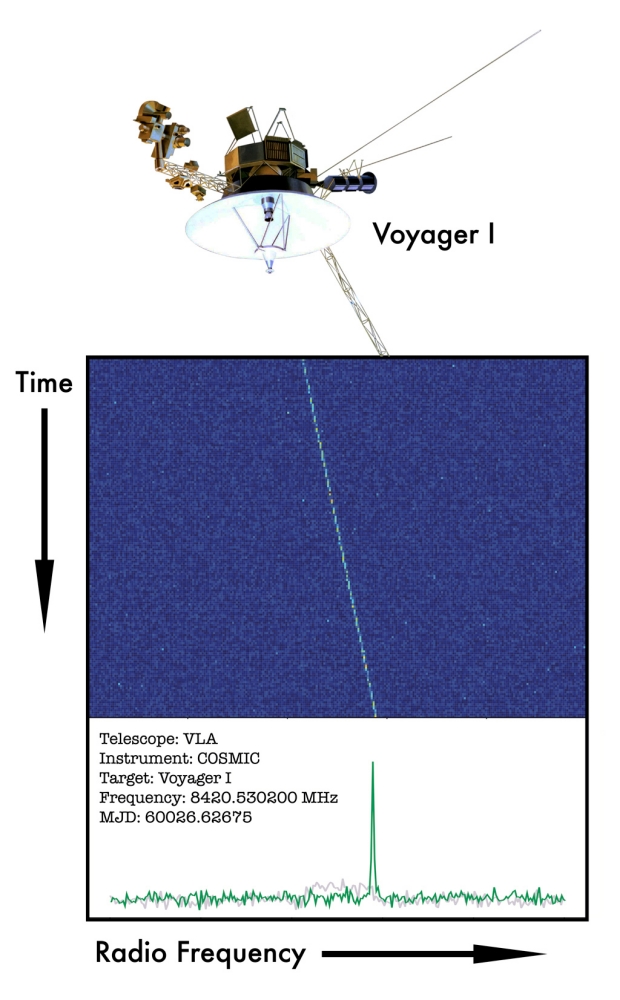
Voyager as Technosignature
Here’s an image that brings out the philosopher in me, or maybe the poet. It’s Voyager 1, as detected by a new processing system called COSMIC, now deployed at the Very Large Array west of Socorro, New Mexico. Conceived as a way of collecting data in the search for technosignatures, COSMIC (Commensal Open-Source Multimode Interferometer Cluster) taps data from the ongoing VLASS (Very Large Array Sky Survey) project and shunts them into a receiver designed to spot narrow channels, on the order of one hertz wide, to spot possible components of a technosignature. Technosignatures fire the imagination as we contemplate advanced civilizations going about their business and the possibility of eavesdropping upon them. But for me, the image below conjures up thoughts of human persistence and a gutsy engagement with the biggest issues we face. Why are we here, and where exactly are we in the galaxy? In the cosmos? Spacecraft like the Voyagers were part of the effort to explore the Solar...
SETI and Signal Leakage: Where Do Our Transmissions Go?
The old trope about signals from Earth reaching other civilizations receives an interesting twist when you ponder just what those signals might be. In his novel Contact, Carl Sagan has researchers led by Ellie Arroway discover an encrypted TV signal showing images from the Berlin Olympics in 1936. Thus returned, the signal announces contact (in a rather uncomfortable way). More comfortable is the old reference to aliens watching “I Love Lucy” episodes in their expanding cone of flight that began in 1951. How such signals could be detected is another matter. I’m reminded of a good friend whose passion for classical music has caused him to amass a collection of recordings that rival the holdings of a major archive. John likes to compare different versions of various pieces of music. How did Beecham handle Delius’ “A Walk in the Paradise Garden” as opposed to Leonard Slatkin? Collectors find fascination in such things. And one day John called me with a question. He was collecting the...
‘Oumuamua: Avi Loeb’s Response to the Molecular Hydrogen Theory
The enigmatic ‘Oumuamua continues to stir controversy. Last week we looked at a new paper from Jennifer Bergner (UC-Berkeley) and Darryl Seligman (Cornell University), discussing a mechanism for the interstellar object’s unusual non-gravitational acceleration. The researchers explored the possibility that ice impacted by high-energy particles like cosmic rays would dissociate water in a comet to create molecular hydrogen within the ice. Was the warming of this hydrogen, all but undetectable according to the authors, the cause of outgassing and the anomalous acceleration? Image: This very deep combined image shows the interstellar object ‘Oumuamua at the center of the image. It is surrounded by the trails of faint stars that are smeared as the telescopes tracked the moving comet. Credit: ESO/K. Meech et al. Answering the question in a paper just submitted to the arXiv site is Harvard’s Avi Loeb, working with Thiem Hoang (Korea University of Science and Technology), who home in on...
MaRMIE: The Martian Regolith Microbiome Inoculation Experiment
Alex Tolley follows up his analysis of agriculture on Mars with a closer look at the Interstellar Research Group’s MaRMIE project – the Martian Regolith Microbiome Inoculation Experiment. Growing out of discussions on methods beyond hydroponics to make the Red Planet fertile, the project is developing an experimental framework, as described below, to test our assumptions about Martian regolith here on Earth. A path forward through simulation and experiment could help us narrow the options for what may be possible for future colonists. Fertile regolith, achieved through perchlorate removal, would open up possibilities far beyond what is achievable through hydroponics. by Alex Tolley Successful settlement of distant locations requires living off the land, which requires resourcing food. Failure can lead to disaster, as experienced by some of the early American colonies. While near Earth space settlements could be supplied with packaged food, this would be too costly for an expanding...
An Appreciation of SETI’s Robert Gray (1948-2021)
Robert Gray was something of an outsider in the community of SETI scientists, spending most of his career in the world of big data, calculating mortgage lending patterns and examining issues in urban planning from his office in Chicago. As an independent consultant specializing in data analysis, his talents were widely deployed. But SETI was a passion more than a hobby for Gray, and he became highly regarded by scientists he worked with, many of whom were both surprised to hear of his death on December 6, 2021. It was Jim Benford who gave me the news just recently, and it humbles me to think that a Centauri Dreams post I worked with Gray to publish (How Far Can Civilization Go?) appeared just months before he died. Gray’s independent status accounts for the lack of publicity about his death in our community, but I’m still startled that I’m only now learning about it. His name certainly has resonance on this site, particularly his book The Elusive Wow: Searching for Extraterrestrial...
Life from Elsewhere
The idea that life on Earth came from somewhere else has intrigued me since I first ran into it in Fred Hoyle's work back in the 1980s. I already knew of Hoyle because, if memory serves, his novel The Black Cloud (William Heinemann Ltd, 1957) was the first science fiction novel I ever read. Someone brought it to my grade school and we passed the copy around to the point where by the time I got it, the paperback was battered though intact. Its cover remains a fine memory. I remember being ingenious about appearing to be reading an arithmetic text in class while actually reading the Hoyle novel. In the book, the approach of a cloud of dust and gas in the Solar System occasions alarm, with projections of the end of photosynthesis as the Sun's light is blocked. Even more alarming are the unexpected movements of the cloud once it arrives, which suggest that it is no inanimate object but a kind of organism. I've been meaning to re-read The Black Cloud for years and this post energizes me...