by Paul Gilster | Mar 4, 2021 | Deep Sky Astronomy & Telescopes |
As we follow the progress of the James Webb Space Telescope through performance tests in preparation for launch, Robert Zubrin has been thinking of far larger instruments. The president of Pioneer Astronautics and founder of the Mars Society thinks we can create telescopes of extremely large aperture — and sharply lower cost — by using the physics of spinning gossamer membranes, a method suitable for early testing as a CubeSat demonstration mission. In today’s essay, Dr. Zubrin explains the concept and considers how best to deploy next generation space telescopes reaching apertures as large as 1000 meters. We can’t know what new phenomena such an instrument would find, but the Enormous Space Telescope fits the theme of breakthrough discovery outlined in his latest book, The Case for Space: How the Revolution in Spaceflight Opens Up a Future of Limitless Possibility (Prometheus, 2020).
by Robert Zubrin
Abstract
This paper presents a method for creating Enormous Space Telescopes (ESTs). The EST employs a hoop to deploy a slack reflector membrane, such as solar sail material or radio dish. When the EST is simultaneously rotated around its center and accelerated along its axis of rotation, the membrane will assume a parabolic shape, thereby creating a reflector for a very large aperture telescope. The EST reflector can be accelerated along its linear axis by tethering its deployment hoop to a tug spacecraft. The tug can exert force on the hoop several methods, including direct thrust, centrifugal rotation of the tethered tug-reflector assembly, or by lowering the reflector from a high altitude balloon or more massive tug positioned in a higher orbit. A force equivalent to linear acceleration can also be generated to shape an EST without a tug using electrostatic means. ESTs can be used for astronomy across a wide spectrum of frequencies, ranging from the ultraviolet, through optical and infrared, down to radio. A demonstration EST with an aperture larger than the Webb Space Telescope could be flown on a CubeSat mission in low Earth orbit. ESTs with apertures of hundreds of meters could be delivered to heliocentric space in single flights of existing launch vehicles.
Background
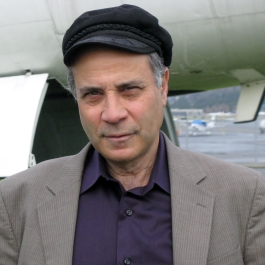
There is no better place to do astronomy than space. Therefore, since the dawn of the space age, it has been the ardent ambition of astronomers to place ever more capable telescopes there. The largest such operational instrument, the 2.4 m diameter aperture Hubble Space Telescope, has benefitted from its location above the Earth’s atmosphere to make many great discoveries, and astronomers hold high hopes for more breakthroughs from the long-awaited 6.5 m diameter Webb Space Telescope. As the light gathering power of a telescope increases with the square of their aperture, still larger space telescopes are greatly to be desired. However, as the cost (>$10 billion) and quarter century long development schedule of the Webb telescope have demonstrated, new techniques will be required if construction of much larger observatories is to be made practical. This is the purpose of the Enormous Space Telescope (EST) concept.
The Enormous Space Telescope (EST)
The EST exploits the principle that if a flexible material subject to a linear acceleration is spun, the balance of linear acceleration and centrifugal acceleration forces will shape the material into a parabolic geometry. This technique has been used on Earth to spin cast liquid glass into parabolic dishes for use in telescopes up to several meters in diameter. The EST can employ similar physics with a properly tailored sheet of gossamer material in space to create parabolic reflector dishes with dimensions of hundreds of meters while keeping system masses well within existing launch vehicle limits.
In order to understand how the EST works, let us start by considering it in its smallest and possibly initial form, as a CubeSat demonstration mission. Consider a 13 kg, 12U CubeSat in a circular orbit 400 km above Earth. A one kilometer long tether is extended down from the satellite, and used to suspend a 13 m diameter (twice that of Webb) hoop, whose central axis aligns with the tether. Lines from the circumference of the hoop attach to the tether by a frictionless magnetic bearing, allowing the hoop to rotate freely. The interior of the hoop contains a slack solar sail material, properly tailored to accept a parabolic shape without folds, which is attached to the hoop like the skin of a slack-topped drum. Aluminized balloon film can be used to create solar sail material with a mass density of 6 grams/m2. Taking the hoop mass into account, we will assume 10 gm/m2 as the net mass density for our hoop/film combination, resulting in a mass estimate of 1.3 kg for that subsystem.
At an altitude of 400 km, the CubeSat will be moving with a velocity of 7668.63 m/s, generating a centrifugal acceleration of 8.6762 m/s2, exactly matching the Earth’s gravitational acceleration at that altitude. The reflector, however, hanging 1 km below the CubeSat will only be moving at 7667.50 km/s, generating a centrifugal acceleration of 8.675 m/s2. The Earth’s gravitational acceleration at that altitude will be 8.679 m/s. Thus the hoop will experience a downward acceleration of 0.004 m/s2, or 0.4 milliGees. This will make the sail film in the hoop sag. But if we rotate the hoop with an edge velocity of 0.1 m/s, the film material will also experience an outward acceleration, ranging from 0 at its center to 0.0015 m/s2 at its edge. Taken in combination with the linear acceleration, this will shape the film into a perfect parabola.

Fig. 1 An EST suspended by a tether in LEO. The telescope parabolic dish is spinning around the axis of the tether. Earth (down) is on the right.
This little demonstration EST, with a total mass less than 20 kg, including optics that would be positioned along or suspended from the tether at the parabola focal point, would have four times the light gathering capacity of Webb (about thirty times that of Hubble), while costing on the order of 1/1000th as much.
An even cheaper flight demonstration could be done suspending an EST from a high altitude balloon. Since a balloon moves with the wind, the payload would feel no wind. At 100,000 ft it would be above 99% of Earth’s atmosphere. A triangle of long spars could be employed with a balloon attached to each vertex, to keep the balloons out of the field of view of the telescope.
Such systems would have limitations, since they would be constantly pointing directly away from the center of the Earth. But we can do better.
Let us therefore scale our unit up in diameter by a factor of ten, to a 130 m diameter reflector dish, increasing the mass of the hoop, the optics and spacecraft by a factor of 100. It would still be a quite manageable mass though, about 2000 kg, easily launchable into interplanetary space by a Falcon 9 medium lift booster. In this case. there would be no gravity gradient available to stretch the tether. So we need to use an alternative technique.
One approach might be to spin the hoop around the spacecraft, in the manner of a tethered artificial gravity system, having a second hoop counter-rotating along with the one suspending the dish in order to neutralize gyroscopic effects. But such a system would still need to constantly change its pointing direction, making long duration exposures impossible.
Tugs for ESTs
A more effective approach would be to simply employ a spacecraft as a tug. Sunlight has a pressure of 9 micronewtons per square meter, which would add up to 0.12 Newtons over the whole body of the 130 m diameter sail. If that were the only linear acceleration of the sail, it would shape it into a parabolic reflector with its concave side pointing towards the Sun. As we want to be able to point the telescope the other way, we need to generate more thrust than that. This could be done using either electric propulsion or larger solar sails with a lower mass density then the hoop, or magnetic or electric sails, pulling its tether outward from the Sun.
Let us first consider electric propulsion. If we had an 70% efficient ion engine using argon propellant and a Isp of 7000 s, 50 kWe would be required to produce 1 N of thrust. Assuming a typical solar electric propulsion system mass to power ratio of 20 kg/kWe, that would require 1000 kg. The tug would thus accelerate at a rate of 0.001 m/s2. If the reflector was made of sail material with the minimum mass density of 6 gm/m2, its material would self-accelerate away from the Sun with an acceleration of 9e-6/0.006 =0.0015 m/s2, which is greater than the self-acceleration of the tug, and therefore unsatisfactory. However, the remedy for this is simple: just make the reflector material much thicker. For example, if we tripled its thickness to 18 gm/m2, its self-acceleration would only be 0.0005 m/s2, i.e. half that of the tug. So it would lag behind the tug and the net pull on it of 0.0005 m/s2 would make its center sag back towards the Sun. If we then set it spinning with a velocity of 0.1 m/s at its edge, an edge centrifugal acceleration of 0.00015 m/s2 would be created, shaping it into a 130 m diameter parabolic dish.

Fig. 2 Electric Propulsion tug pulling on an EST.
Operating at 1 N thrust, the thruster would consume 0.014 gm/s of propellant, or about 1.2 kg per day of thrusting (i.e. observing time). Thrust and thus propellant requirements would drop if the telescope were positioned further out in the solar system, since solar light pressure would drop as the inverse square of the telescope’s distance from the Sun. Thus, for example at 3.1 AU, it would only need to use 0.12 kg/day of propellant to generate adequate acceleration.
We could also use solar sails as tugs. In this case no propellant would be needed. Positioning the tug behind the EST would allow it to eclipse solar pressure, as shown if Fig. 3. If the tug is pulling the EST, making tug acceleration greater than reflector material self-acceleration could be assured simply by having the tug sails be larger than the reflector sail, and using a heavy gauge material for the reflector sail.
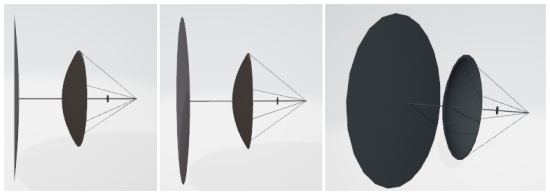
Fig. 3 Using solar sail tugs to accelerate an EST, by pushing from behind. The EST spins around the central axis. The Sun is on the left. An alternative design would send the mast through the sunward pusher sail, allowing it to deliver its thrust to the base of the mast by a set of shrouds.
A pusher sail telescope would need to point (generally, but not necessarily exactly) outward from the Sun all the time. However if a nuclear electric tug were used, and the telescope were positioned in the shadow of a planet, sunlight impinging on the rear side of the reflector would not be an issue and the telescope could be pointed in any direction.
In the case of radio telescopes, all of this becomes much easier, as there would be no solar light pressure on the rear face of the dish. In that case any kind of tug – solar electric, nuclear electric, or solar sail- could be used, with the EST pointable in any direction simply by maneuvering the tug. The amount of acceleration required from the tug could also be much less.
The Electrostatic EST
An alternative to physical acceleration to impose linear force on the dish is to use electrostatic attraction, In this case the reflector sail would be charged one way, while another sail positioned behind it and held off at a distance by a structural system would be given an opposite charge. The sails would thus attract each other, much as if by gravity, and when the assembly was spun up, both sails would assume parabolic shapes, with their concave sides pointing in opposite directions.
Let us consider the case of two 50 m radius dishes held 25 m apart by structure, with a potential difference between the two of 10 kV, creating a field of 400 volts/m. From electrostatics we have EA = Q/?, so Q, the charge on each dish will be given by Q=(400)(7854 m2)(8.85e-12) = 2.8e-5 coulombs. The electrostatic force on each sail will be given by F=QE, so the total electrostatic force between the sails will be F=400(2.8e-5) = 0.0112 N. Assuming the sail materials have a mass density of 6 gm/m2, this will result in a self-acceleration each sail towards the other of 0.0112/(0,006)(7854) = 0.00024 m/s2. It may be observed that the field will actually be greater near the center because the dishes would sag towards each other. This, however, could be compensated for by varying the thickness of the sail material, making it thicker towards the center and thinner towards the edge, thereby keeping the linear self-acceleration of the two sails towards each other equal over their entire surfaces.
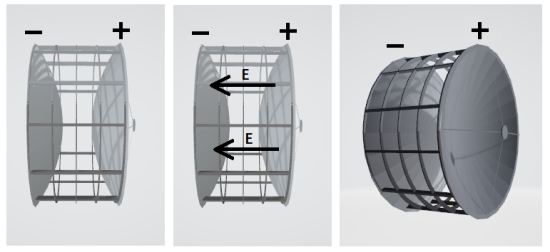
Fig. 4. An Electrostatic EST. The sails have opposite charges and are held separate from each other by a compressive structure. The mutual attraction of the sails can substitute for linear acceleration of the system
Size Limits of ESTs
There does not seem to be any theoretical limit to the potential size of an EST. However, as we have seen, using current materials, the mass required the create an EST system goes approximately as:

Where M is the EST system mass in kilograms and R is the aperture radius in meters. Thus our 6.5 m radius EST demo unit has an estimated mass, including its associated spacecraft, on the order of 20 kg, while our 65 m radius operational EST would be expected to have a mass on the order of 2000 kg.
Currently the largest operational launch vehicle is the Falcon Heavy, with a capability of about 60,000 kg to low Earth orbit (LEO). More powerful vehicles, including the NASA SLS and the SpaceX Starship system are expected to become operational within the next few years, with capabilities of up to 120,000 kilograms to LEO. Since an EST tug could propel itself out of LEO and into heliocentric space, this may also be taken as the limit of the size of an EST system, deliverable into space with a single launch. If we plus 120,000 kg into equation (1), we find that a practical size limit for relatively near-term EST systems would be an aperture diameter of about 1000 meters. The discoveries that might be enabled by such systems are beyond reckoning.
Conclusion
We find that the EST concept offers a practical path towards creating space telescopes with capabilities dwarfing conventional systems by many orders of magnitude. We also find that ESTs could be used to create space telescopes with comparable capabilities to conventional systems, but with several orders of magnitude lower cost. Furthermore, the EST concept holds these benefits for space astronomy across a wide range of frequencies, from ultraviolet down to radio. We therefore recommend that the concept be studied further, and that a demonstration mission be flown at an early date.
Acknowledgement; The author wishes to acknowledge the assistance of Heather Rose, who provided the illustrations for this paper.
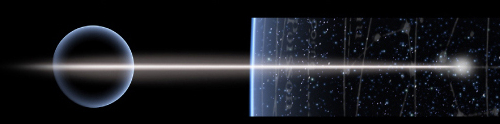
by Paul Gilster | Jan 28, 2021 | Deep Sky Astronomy & Telescopes |
This seems to be the week of unusual configurations. Following up on TRAPPIST-1 and TOI-178 comes TYC 7037-89-1, where we have fully six stars in a single system, all of which participate in eclipses. In other words, what TESS has revealed is a system consisting of three eclipsing binaries. It’s located about 1,900 light years out in Eridanus, and if it doesn’t remind you of Isaac Asimov’s “Nightfall,” nothing will. In the story (published in the September 1941 issue of Astounding Science Fiction), the planet Lagash is illuminated almost constantly by one of the six stars in its system. The discovery of what happens when it is not — which occurs every 2,000 years or so — drives the plot of one of Asimov’s best tales.
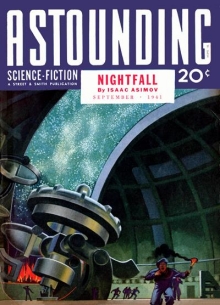
TYC 7037-89-1 (also known as TIC 168789840), marks the first time a six-star system has been found where all the stars are involved in eclipses as seen from our vantage point. This leads to complicated orbital dynamics. With the three binaries designated A, B and C, we learn that the two stars making up A and C respectively orbit each other every day and a half, as measured by the dip in system brightness when one star passes in front of another. The A and C binaries, in turn, orbit each other on a timeframe of about four years.
But we’re not through. The binary B also has two members, which orbit each other every eight days. This pair is considerably further away, so that it orbits the two inner binaries every 2,000 years or so (there goes Asimov again). All three binaries have primary stars a bit larger and more massive than the Sun, with the secondary stars in all three cases being about half the Sun’s size, and no more than a third as hot. The remarkable point is that to detect all three binaries, we have to have orbital planes aligned with our observations from TESS, and this is occurring despite their wide separation. Have a look at all this in the diagram below.
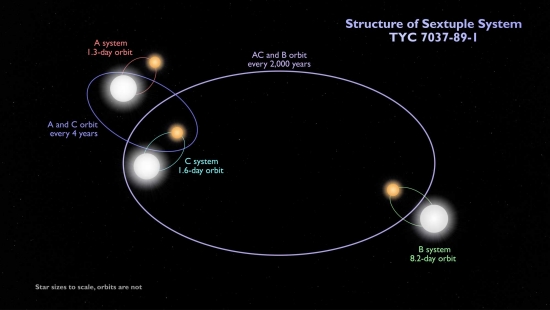
Image: This schematic shows the configuration of the sextuple star system TYC 7037-89-1. The inner quadruple is composed of two binaries, A and C, which orbit each other every four years or so. An outer binary, B, orbits the quadruple roughly every 2,000 years. All three pairs are eclipsing binaries. The orbits shown are not to scale. Credit: NASA’s Goddard Space Flight Center.
Veselin Kostov (SETI Institute) and Brian Powell (NASA GSFC) led the team behind this discovery. Says Kostov:
“Multiply-eclipsing multiple systems such as TYC 7037-89-1 enable simultaneous, precise measurements on the stellar sizes, temperatures, and potentially masses, of pairs of stars that share common history. In turn, this provides better understanding of stellar formation and evolution in dynamically-rich environments.”
The international team included Saul Rappaport (MIT), Tamás Borkovits (University of Szeged, Hungary), Petr Zasche (Charles University, Czech Republic, and Andrei Tokovinin (NSF NOIRLab). A great deal of data mining went into their study, which included using the NASA Center for Climate Simulation’s Discover supercomputer at GSFC to examine brightness variations in 80 million stars in the TESS dataset. Their neural network found 450,000 eclipsing binary candidates, about 100 of which included three or more stars, as well as this system.
TYC 7037-89-1 naturally raises questions about how such a system formed and provides a useful laboratory for the study of orbital interactions in such complex scenarios. And while it’s not the first six-star system we’ve found, it’s the first to show three eclipsing binaries. From the paper:
TIC 168789840 is a fascinating system that naturally merits additional observation and analysis. Though quite similar to the famous Castor system, the “triplet” nature of TIC 168789840 combined with the presence of three primary and three secondary eclipses enable further investigations into its stellar formation and evolution. Remarkable objects like TIC 168789840 or Castor give us insights on the formation of multiple systems — a matter of active research and debate. It is well known that components of hierarchical systems have correlated masses (Tokovinin 2018a), suggesting accretion from a common source. On the other hand, disk fragmentation and subsequent migration, driven by accretion, appears to be the dominant mechanism of close binary formation…
One possible model discussed in the paper: A binary star captures a third star, with subsequent fragmentation of each of the stars:
With regard to TIC 168789840, we might think that an encounter of the young binary AC with another star B led to its capture on a wide orbit, while strong accretion from the unified envelope, caused by this dynamical event, formed seed secondary companions to all stars by disk fragmentation. The seeds continued to grow and migrate inward, while the intermediate and outer orbits also evolved.
That, of course, is one among a cluster of possibilities to be investigated as more candidate multi-star systems are analyzed. The paper points out that another sextuple system is to be analyzed in a forthcoming paper, which will make a useful point of comparison. Meanwhile, we’re reminded again of the power of artificial intelligence at producing discoveries in voluminous datasets. AI is increasingly the pointer for follow-up studies that pay off.
The paper is Powell et al., “TIC 168789840: A Sextuply-Eclipsing Sextuple Star System,” accepted at The Astronomical Journal (preprint).
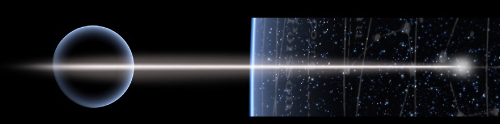
by Paul Gilster | Jan 14, 2021 | Deep Sky Astronomy & Telescopes |
Something to note about the brown dwarfs we looked at yesterday: Our views on how they would appear to someone nearby in visible light are changing. It’s an interesting issue because these brown dwarfs exist in more than a single type. If you’ll have a look at the image below, you’ll see a NASA artist conception of the three classes of brown dwarf, all of these being objects that lack the mass to burn with sustained fusion.
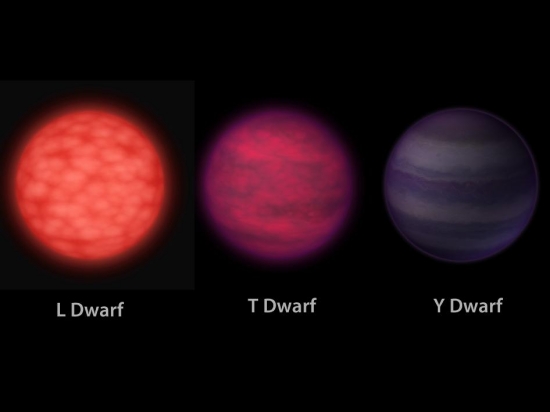
Image: This artist’s conception illustrates what brown dwarfs of different types might look like to a hypothetical interstellar traveler who has flown a spaceship to each one. Brown dwarfs are like stars, but they aren’t massive enough to fuse atoms steadily and shine with starlight — as our sun does so well. Our thoughts on how these objects appear are evolving quickly, as witness yesterday’s discussion, and we’re likely to need another visual rendering of brown dwarf classes soon. Credit: NASA/JPL-Caltech.
One thing should jump out to anyone who read yesterday’s post on the appearance of Luhman 16 B: The artist here does not depict bands of clouds/weather on the object, but rather localized storms of the kind that some researchers believed would characterize brown dwarfs. We know that Luhman 16 A (33 times Jupiter’s mass) is of spectral type L7.5, while Luhman 16 B is categorized as T0.5, putting it near the transition between types L and T. And Luhman 16 B shows strong evidence of banding.
That’s according to Daniel Apai and team, as discussed yesterday, in an analysis based on data from TESS. Looking further at the image above, it’s clear we’re going to be re-working our depictions going forward as we analyze more brown dwarfs. If we should expect a banded object at the L-T transition, then at least the L dwarf and the T dwarf shown here will likely show the same atmospheric pattern (obviously, we’ll need to confirm these speculations with hard data). That would leave the Y dwarf as yet undetermined, and for good reason, as these objects are vanishingly hard to see.
Atmospheric temperatures drop as we move across the types of brown dwarfs here, with the L dwarf being the brightest and hottest in the image; its typical temperatures are in the range of 1400 degrees Celsius. The magenta T dwarf takes us down to about 900 degrees Celsius, but the Y dwarf really drops the reading, with the coldest yet identified having a temperature of a mere 25 degrees Celsius. That’s not all that far off what my thermostat is set on — 72 ? — as I try to take the chill off this morning.
All three of the brown dwarfs shown above appear at the same size, a reminder that all types of this object have the same dimension, which is roughly that of Jupiter, despite wide variations in their mass. Same radius, major disparity in mass, in other words. My hopes that we would find one of these fascinating objects at no more than, say, 1 light year seem to have been dashed, although it’s certainly true that Y dwarfs are so cool that finding them is going to be difficult even for the best infrared observatories.
As we keep looking, we can now refer to the updated map of L, T and Y dwarfs in the vicinity of the Solar System that the Backyard Worlds: Planet 9 project has produced. You’ll recall from earlier posts here that Backyard Worlds: Planet 9 is funded by NASA as a collaboration between professional scientists and the public.
All those non-professional but often highly adept astronomers and volunteers have produced a map with a radius of about 65 light years. The work of 150,000 volunteers has been going on since 2017 using data from the WISE mission under its Near-Earth Object Wide-Field Infrared Survey Explorer (NEOWISE) incarnation. The study was presented at the ongoing virtual meeting of the American Astronomical Society.
Dozens of new brown dwarfs turned up in this work, which drew on data from the now retired Spitzer Space Telescope. Using the Backyard Worlds: Planet 9 results, astronomers consulted data from the space telescope to observe 361 local brown dwarfs of types L, T and Y and combined the results with previously known dwarfs, many of them catalogued by CatWise, the catalog of objects from WISE and NEOWISE.
The result: a 3D map of 525 brown dwarfs.
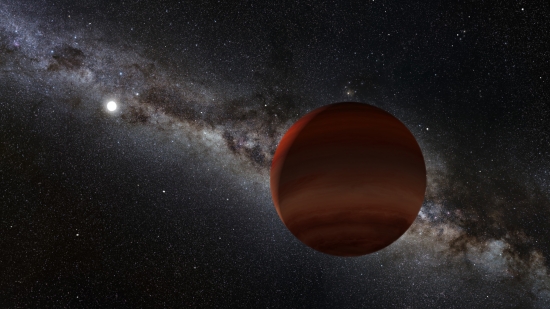
Image: In this artist’s rendering, the small white orb represents a white dwarf (a remnant of a long-dead Sun-like star), while the purple foreground object is a newly discovered brown dwarf companion, confirmed by NASA’s Spitzer Space Telescope. This faint brown dwarf was previously overlooked until being spotted by citizen scientists working with Backyard Worlds: Planet 9, a NASA-funded citizen science project. Credits: NOIRLab/NSF/AURA/P. Marenfeld/Acknowledgement: William Pendrill.
The galaxy’s coldest known Y dwarf is a neighbor (not surprising, given that more distant dwarfs should be below the level of detection), but it turns out that it is comparatively rare, a bit of an anomaly given our expectations of brown dwarf distribution. Of the seven objects nearest to our Solar System, three are brown dwarfs. And the Sun’s position within this cluster of nearby objects is a bit unusual as well, says Aaron Meisner (National Science Foundation NOIRLab), a co-author of the study:
“If you were to put the Sun at a random place within our 3D map and you were to ask, ‘Typically, what do its neighbors look like?’ We find that they would look very different from what our actual neighbors are.”
Again, we have to weigh this outcome against the difficulty in observing Y dwarfs, so conclusions shouldn’t be drawn too hastily. With brown dwarfs having exoplanet dimensions but no companion main sequence star (in most cases), they become useful objects as we refine the tools of exoplanet characterization. The James Webb Space Telescope should be able to tell us more about nearby brown dwarfs, as will the upcoming SPHEREx mission, an all-sky infrared survey scheduled for a 2024 launch.
The paper is Marocco et al., “The CatWISE2020 Catalog,” accepted for publication in the Astrophysical Journal Supplement Series (abstract/preprint).
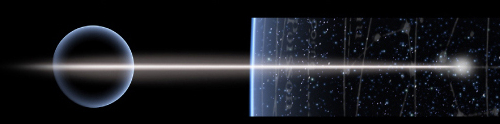
by Paul Gilster | Jan 13, 2021 | Deep Sky Astronomy & Telescopes |
I keep hoping we’ll find a brown dwarf closer to us than Alpha Centauri, but none have turned up yet despite the best efforts of missions like WISE (Wide-Field Infrared Survey Explorer). If there’s something out there, it’s dim indeed. Of course, I wouldn’t be surprised at finding rogue planets between us and the nearest stars. Maybe some will be more massive than Jupiter, but evidently not massive enough to throw an infrared signature of the sort that defines a brown dwarf. Just what lies outside our system’s edge always makes for interesting speculation.
The beauty of finding an actual brown dwarf as opposed to a rogue planet is that we might be dealing with a planetary system in miniature, a fine target in our own backyards. Lacking that, the closest brown dwarf we know is the Luhman 16 AB system, a binary in the southern constellation of Vela some 6.5 light years from the Sun (a little further than Barnard’s Star, making this the third closest known system to the Sun). Here we have one dwarf about 34 times Jupiter’s mass (Luhman 16 A), and another, Luhman 16 B, about 28 times more massive than Jupiter, and because both are brown dwarfs, both are hotter than the planet.
Luhman 16 AB is the subject of a new paper from Daniel Apai (University of Arizona / Lunar and Planetary Laboratory). Apai’s team was intent on finding out what brown dwarfs look like, wondering whether they’d be marked by the kind of well-defined banding and belts we see on Jupiter or roiling with storms of the kind we’ve seen (thanks to Juno) on Jupiter’s poles. The method: Using data from TESS (Transiting Exoplanet Survey Satellite), the researchers deployed in-house algorithms to measure brightness changes of the two brown dwarfs as they rotated. Brighter atmospheric features rotate in and out of view.
What emerged was the most detailed look yet at a brown dwarf’s atmospheric circulation, and that led to conclusions about the appearance of these objects. We now know that Jupiter is a good analogy for what we would see if we could look at Luhman 16 AB up close. The work created a model for Luhman 16 B’s atmosphere showing that high-speed winds run parallel to the brown dwarf’s equator. Also like Jupiter are the apparent vortices emerging in the polar regions. Here we need to pause to thank the late Adam Showman, also of the University of Arizona, whose models predicted this pattern.
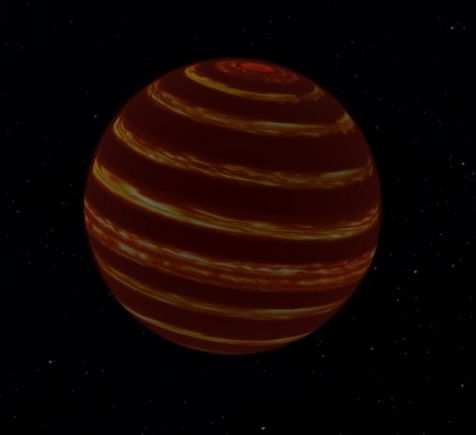
Image: Using high-precision brightness measurements from NASA’s TESS space telescope, astronomers found that the nearby brown dwarf Luhman 16 B’s atmosphere is dominated by high-speed, global winds akin to Earth’s jet stream system. This global circulation determines how clouds are distributed in the brown dwarf’s atmosphere, giving it a striped appearance. Credit: Daniel Apai.
The lighter zones shown above are thought to be thin cloud decks illuminated by light from the hot interior, while the darker zones are where thicker cloud decks block interior light. The wind speeds are highest at the equator, dropping at the higher latitudes. The global wind pattern is lost at the poles, which are a region of enormous local storms, as on Jupiter. Most of Luhman 16 B, then, is dominated by global wind patterns rather than localized storms.
Something of a surprise to the team (the paper refers to the development as ‘a stunning feature’) is the changeable, non-periodic nature of the Luhman 16 light curve. Here’s how the authors describe this fact:
…we identify four properties that are shared between the visual lightcurve of this object and the infrared lightcurves of other objects: 1) The lightcurves remain variable over long periods (years); 2) The lightcurve shape evolves, yet it displays characteristic period, which is likely the rotational period of the object (as found in Apai et al. 2017); 3) In spite of the rapid evolution of the lightcurve, the amplitudes over rotational time-scales remain similar and characteristic to the object; 4) The lightcurves tend to be symmetric in the sense of similar amount of positive-negative features, in contrast to, for example, a situation in which a single positive feature appears periodically on an otherwise flat lightcurve, which would indicate a single bright spot in the atmosphere.
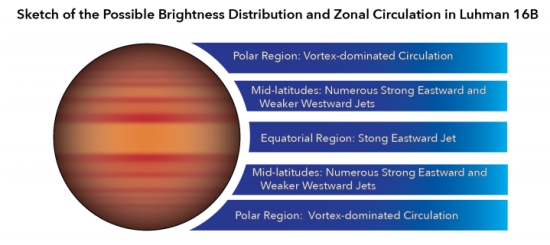
Image: This is Figure 16 from the paper. Caption: Sketch of the possible appearance of Luhman 16B, based on the emerging evidence. Zonal circulation models and comparison to Jupiter suggests that low-latitude regions are dominated by the fastest jets, and that wind speeds at mid-latitude are significantly lower. Circulation at the polar regions is likely to be vortex- and not jet-dominated. Cloud cover is likely to be correlated with the atmospheric circulation. Credit: Apai et al.
All this is drawn from TESS lightcurves of Luhman 16 AB covering 22 days and 100 rotations of the binary, allowing the researchers to conclude that both the brown dwarfs in this system show zonal circulation and fit the Jupiter model. It seems apparent that brown dwarfs can serve as more massive analogs of giant exoplanets and could thus help us develop techniques of atmospheric analysis that can be deployed even further from the Solar System. Says Apai:
“No telescope is large enough to provide detailed images of planets or brown dwarfs. But by measuring how the brightness of these rotating objects changes over time, it is possible to create crude maps of their atmospheres – a technique that, in the future, could also be used to map Earthlike planets in other solar systems that might otherwise be hard to see… Our study provides a template for future studies of similar objects on how to explore – and even map – the atmospheres of brown dwarfs and giant extrasolar planets without the need for telescopes powerful enough to resolve them visually.”
The paper is Apai et al. “TESS Observations of the Luhman 16 AB Brown Dwarf System: Rotational Periods, Lightcurve Evolution, and Zonal Circulation,” Astrophysical Journal Vol. 906, No. 1 (7 January 2021). Abstract / preprint.
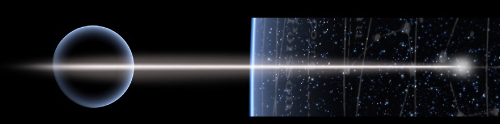
by Paul Gilster | Nov 24, 2020 | Deep Sky Astronomy & Telescopes |
The evolving system known as [BHB2007] 1 is a part of the Pipe Nebula (also called Barnard 59), about 600 light years away in the constellation Ophiuchus. It is part of a binary star system in formation that has been studied with the Atacama Large Millimeter Array (ALMA). Both protostars show disks in formation around them, surrounded by filaments of gas and dust drawn from the larger disk that are being referred to as ‘feeding filaments.’ Paola Caselli (Max Planck Institute for Extraterrestrial Physics, Germany), who made that reference in 2019, is co-author of new work on the stellar object, which gives us an unusual look at early system formation.
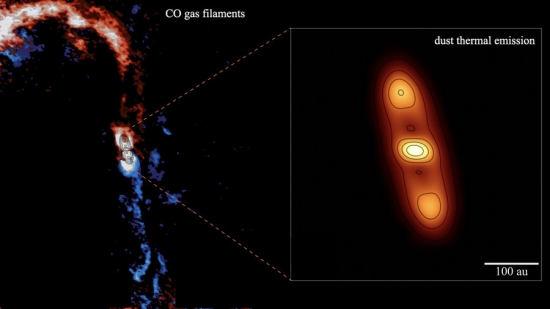
Image: This false-color image shows the filaments of accretion around the protostar [BHB2007] 1. The large structures are inflows of molecular gas (CO) nurturing the disk surrounding the protostar. The inset shows the dust emission from the disk, which is seen edge-on. The “holes” in the dust map represent an enormous ringed cavity seen sideways in the disk structure. Credit © MPE.
This is apparently a case of star and planet growing in tandem. The young stellar object [BHB2007] 1 is thought to be no older than 1 million years, with most of the surrounding envelope having dissipated and clear emission visible from the circumstellar disk. The authors note the clean, wide gap in the dust, adding that it is surprising for such a young system, for accretion from the molecular cloud seems to be continuing, as the filament activity makes clear.
Most circumstellar disks in this age range are fully formed as planet formation begins. Felipe Alves (MPE) is lead author on the paper, which has just appeared in Astrophysical Journal Letters:
“We were quite surprised to observe such prominent accretion filaments falling into the disk. The accretion filament activity demonstrates that the disk is still growing while simultaneously nurturing the protostar.”
Supplementing the ALMA data with observations at radio frequencies from the Very Large Array, the researchers make the case for a young giant planet or perhaps a brown dwarf present within a 70 AU cavity inside the disk, a zone filled with hot molecular gas. Planetary accretion of an object between 4 and 70 Jupiter masses would produce the disk gap.
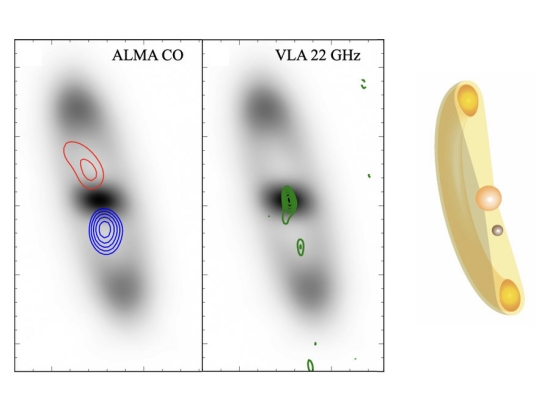
Image: Two different observations of the protoplanetary disk show signatures of the formation of a companion to the protostar . The grey scale represents the dust thermal emission from the disk, same as in the inset of Fig. 1. The red/blue contours show the molecular CO brightness emission levels from the northern/southern side of the dust cavity observed with ALMA. The brighter CO emission from the south indicates that the gas is hotter there. This location coincides with a zone of non-thermal emission tracing ionised gas (green contours) observed with the VLA (middle), which is observed in addition to the protostar (centre of the image). The team proposes that both the ionised gas and the hot molecular gas are due to the presence of a protoplanet or a brown dwarf in the cavity. The configuration of such a system is shown in the sketch on the right. Credit: © MPE; illustration: Gabriel A. P. Franco.
MPE’s Caselli explains the significance of the finding:
“We present a new case of star and planet formation happening in tandem. Our observations strongly indicate that protoplanetary disks keep accreting material also after planet formation has started. This is important because the fresh material falling onto the disk will affect both the chemical composition of the future planetary system and the dynamical evolution of the whole disk.”
Studying star and planet formation together gives us interesting constraints for how young systems evolve out of the original cloud surrounding them. [BHB2007] 1 gives us the kind of gap in a circumstellar disk we’ve observed in other young systems, but in this case it’s a disk still being actively fed by filaments from the surrounding molecular cloud. In at least one case, then, we see the possible formation of planets before the disk itself has fully formed. The existence of an object in formation is supported by VLA’s data on radio emission within the dust gap between the inner and outer disk, giving heft to the assertion that simultaneous formation is occurring.
The paper is Alves et al., “A case of simultaneous star and planet formation,” Astrophysical Journal Letters Vol. 904, No. 1 (19 November 2020). Abstract / Preprint.
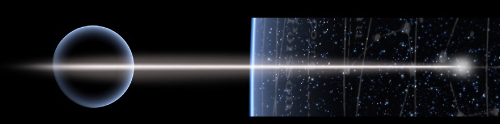
by Paul Gilster | Nov 6, 2020 | Deep Sky Astronomy & Telescopes |
A sequence of new observations gives us a leading candidate to explain Fast Radio Bursts (FRBs). These powerful bursts of radio waves, lasting but milliseconds, first turned up in our data in 2007 and have been a mystery ever since. As they were found in other galaxies, it has been difficult to determine their exact location, and they were impossible to predict as most seemed to be one-off events, although astronomers have subsequently found some that do repeat.
Among the possible causes of FRBs, stellar remnants have been put forward, with the kind of highly magnetic neutron stars called magnetars receiving close scrutiny because their magnetic fields could be the engine driving the bursts. We now have three papers in Nature that give us tight observational evidence of the kind that has been lacking. Between the three, we have data that for the first time link an FRB in our own galaxy to a magnetar, the object known as SGR 1935+2154, located in the constellation Vulpecula.
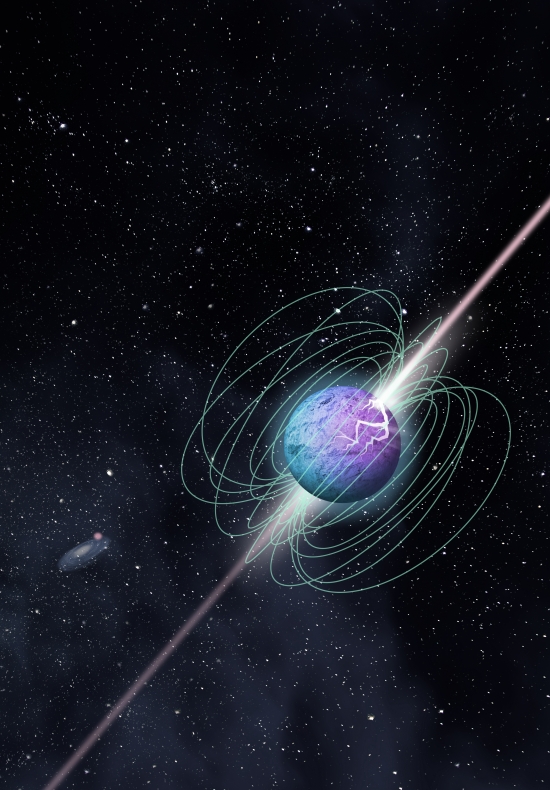
Image: Artist’s impression of a magnetar in outburst, showing complex magnetic field structure and beamed emission, here imagined as following a crust cracking episode. Credit: McGill University Graphic Design Team.
Let’s look at the events involved here. We begin with two space-based observatories. Both the Fermi Gamma-ray Space Telescope and the Neil Gehrels Swift Observatory registered rapid bursts of gamma- and X-rays from this object in late April. The spate of burst activity lasted for hours and was also observed by NASA’s Neutron star Interior Composition Explorer, an X-ray telescope mounted on the International Space Station.
This was followed by observations about thirteen hours later of another X-ray burst, once again seen by numerous instruments, including the European Space Agency’s INTEGRAL mission as well as China’s Huiyan X-ray satellite and the Russian Konus gamma-ray burst monitor, a Russian experiment that flies aboard NASA’s GGS-Wind spacecraft. This was a burst lasting about half a second, but as it flared, the Canadian Hydrogen Intensity Mapping Experiment (CHIME) and the Survey for Transient Astronomical Radio Emission 2 (STARE2), located at CalTech, detected a Fast Radio Burst (FRB 200428) in the same part of the sky.
Scientists at the Chinese Five-hundred-meter Aperture Spherical radio Telescope (FAST) had been observing SGR 1935+2154 for some weeks. As I understand it, they did not see FRB 200428, but were subsequently able to detect an FRB at the same location (FAST was not observing the object when FRB 200428 was detected by the other instruments).
Citations for papers from both the CHIME/FRB Collaboration and the STARE2 team are given below, along with a citation for a paper from FAST astronomers that provides limits to the radio flux of the FRB. The authors of the latter find a ‘weak correlation’ between FRBs and SGR 1935+2154, as co-author Zhang Bing (University of Nevada) explains:
“The weak correlation could be explained by special geometry and/or limited bandwidth of FRBs. The observations of SGR J1935 start to reveal the magnetar origin of FRBs, although other possibilities still exist.”
So we have a burst of X-rays associated with an FRB, the latter radio component detected first at CHIME and then STARE2. Moreover, the radio burst from SGR 1935+2154 was thousands of times brighter than any radio emissions previously observed from magnetars in the Milky Way, and in fact would have registered as a weak FRB had it occurred in another galaxy. The arrival of the radio pulse during an X-ray burst points to this magnetar as the source.
Paul Scholz is a researcher at the University of Toronto’s Dunlap Institute for Astronomy & Astrophysics and a member of the CHIME/FRB Collaboration:
“The radio burst was far brighter than anything we had seen before, so we immediately knew it was an exciting event. We’ve studied magnetars in our galaxy for decades, while FRBs are an extragalactic phenomenon whose origins have been a mystery. This event shows that the two phenomena are likely connected.”
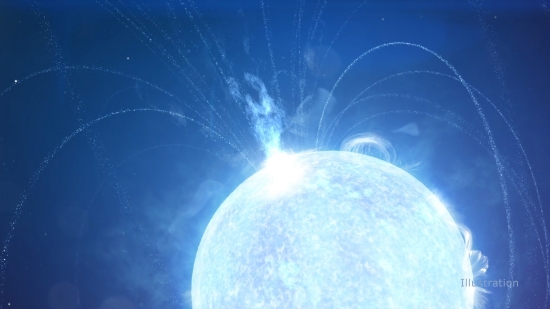
Image: A powerful X-ray burst erupts from a magnetar – a super-magnetized version of a stellar remnant known as a neutron star – in this illustration. A radio burst detected April 28 occurred during a flare-up like this on a magnetar called SGR 1935. Credit: NASA’s Goddard Space Flight Center/Chris Smith (USRA).
The magnetic field of a magnetar can be a thousand times stronger than that of a typical neutron star, offering us a generous source of power, but at the same time, the radio burst from SGR 1935+2154 was thousands of times brighter than any radio emissions ever detected from magnetars in our galaxy. Paul Scholz has speculated that it may require the youngest, most active magnetars to explain the range of FRB sources we’ve thus far observed elsewhere, although we have yet to make a simultaneous detection tying an FRB with an X-ray burst in another galaxy, a signature that efforts like CHIME will continue to look for.
George Younes (George Washington University) is lead author on two upcoming papers on the FRB. Here he speculates on the nature of the burst activity:
“The bursts seen by NICER and Fermi during the storm are clearly different in their spectral characteristics from the one associated with the radio blast. We attribute this difference to the location of the X-ray flare on the star’s surface, with the FRB-associated burst likely occurring at or close to the magnetic pole. This may be key to understanding the origin of the exceptional radio signal.”
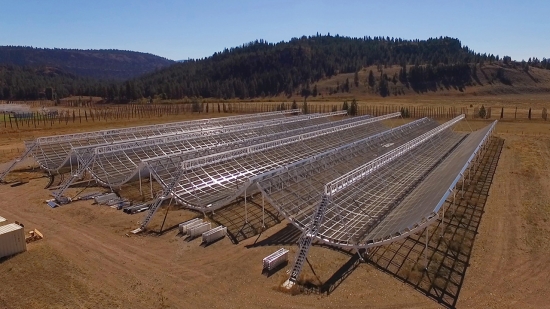
Image: This aerial view shows the Canadian Hydrogen Intensity Mapping Experiment (CHIME), a radio telescope located at Dominion Radio Astrophysical Observatory in British Columbia. Credit: Richard Shaw/UBC/CHIME Collaboration.
So we have the first FRB found in the Milky Way, one whose association with a magnetar may help us place these bursts in context now that we know magnetars can drive FRB activity. On exactly how a magnetar produces an FRB, I want to quote Amanda Weltman and Anthony Walters, who provide a fine overview of this work in an article in Nature that is not behind the journal’s firewall:
…there are several mechanisms by which magnetars can drive FRBs, each of which has a distinct observational signature. The new results thus open up a host of exciting problems to explore. For example, what theoretical mechanism could give rise to such bright, yet rare, radio bursts with X-ray counterparts? One promising possibility is that a flare from a magnetar collides with the surrounding medium and thereby generates a shock wave. Observations of nearby rapidly star-forming galaxies will be crucial for finding events similar to FRB 200428, to help pin down the actual mechanism.
The paper from the STARE team is Bochenek et al., “A fast radio burst associated with a Galactic magnetar,” Nature 587 (2020), pp. 59-62 (abstract). The CHIME/FRB paper is “A bright millisecond-duration radio burst from a Galactic magnetar,” Nature 587 (2020), pp. 54-58 (abstract). The Lin paper is “No pulsed radio emission during a bursting phase of a Galactic magnetar,” Nature 587 (2020), pp. 63-65 (abstract).
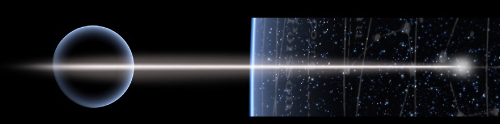