Even as I’ve been writing about the need to map out regions just outside the Solar System, I’ve learned of a new study that finds (admittedly scant) evidence for a Planet 9 candidate. I won’t get into that one today but save it for the next post, as we need to dispose of the New Horizons news first. But it’s exciting that a region extending from the Kuiper Belt to the inner Oort is increasingly under investigation, and the very ‘walls’ of the plasma bubble within which our system resides are slowly becoming defined. And if we do find Planet 9 some time soon, imagine the focus that will bring to this region. As to New Horizons, there are reasons for building spacecraft that last. The Voyagers may be nearing the end of their lives, but given that they were only thought to be operational for a scant five years, I’d say their 50-year credentials are proven. And because they had the ability to hang in there, they’ve become our first interstellar mission, still reporting data,...
Can an Interstellar Generation Ship Maintain a Population on a 250-year Trip to a Habitable Exoplanet?
I grew up on generation ship stories. I'm thinking of tales like Heinlein's Orphans of the Sky, Aldiss' Non-Stop, and (from my collection of old science fiction magazines) Don Wilcox's "The Voyage that Lasted 600 Years." The latter, from 1940, may have been the first generation ship story ever written. The idea grows out of the realization that travel to the stars may take centuries, even millennia, and that one way to envision it is to take large crews who live out their lives on the journey, their descendants becoming the ones who will walk on a new world. The problems are immense, and as Alex Tolley reminds us in today's essay, we may not be fully considering some of the most obvious issues, especially closed life support systems. Project Hyperion is a game attempt to design a generation ship, zestfully tangling with the issues involved. The Initiative for Interstellar Studies is pushing the limits with this one. Read on. by Alex Tolley "Space," it says, "is big. Really big. You...
Deep Space Implications for CubeSats
The Hera mission has been dwarfed in press coverage by the recent SpaceX Starship booster retrieval and the launch of Europa Clipper, both successful and significant. But let’s not ignore Hera. Its game plan is to check on the asteroid Dimorphos, which became the first body in the Solar System to have its orbit altered by human technologies when the DART spacecraft impacted it in 2022. Hera is all about assessing this double asteroid system to see first-hand the consequences of the impact, which shortened the smaller object’s orbit around asteroid Didymos by some 32 minutes. That’s a pretty good result, some 25 times what NASA had defined as the minimum successful orbital period change, and we’re learning more about the ejecta, which involve tons of asteroidal rock. The collision occurred at 6.1 kilometers per second, to be more fully assessed by Hera’s twin CubeSat craft, which will make precise measurements of Dimorphos’ mass to analyze the efficiency of the impact. All this...
Voyager 1: A Splendid Fix
Although it’s been quite some time since I’ve written about Voyager, our two interstellar craft (and this is indeed what they are at present, the first to return data from beyond the heliosphere) are never far from my mind. That has been the case since 1989, when I stayed up all night for the Neptune encounter and was haunted by the idea that we were saying goodbye to these doughty travelers. Talk about naivete! Now that I know as many people in this business as I do, I should have realized just how resilient they were, and how focused on keeping good science going from deep space. Not to mention how resilient and well-built the craft they control are. Thirty five years have passed since the night of that encounter (I still have VCR tape from it on my shelf), and the Voyagers are still ticking. This despite the recent issues with data return from Voyager 1 that for a time seemed to threaten an earlier than expected end to the mission. We all know that it won’t be all that long before...
Deep Space Trajectories: Exiting the Heliosphere
Eugene Parker, after whom the Parker Solar Probe was named, seems to have been the first to have accurately predicted the stream of particles emitted by the Sun that forms the ‘solar wind.’ Parker made the call in a 1958 paper, when solar sailing was just being noised about for the first time, so it wouldn’t have struck him that the term was a bit incautious. Today, when solar sailing is operational, people often assume the solar wind drives solar sails, when in fact the operating principle for solar sails is the momentum generated by photons, which are themselves massless. But streaming particles are indeed a kind of ‘wind,’ and there are magnetic sail concepts tailored for them too. As always, we have to be careful about terminology, especially given the significance of the solar wind in defining our Solar System’s environment. Solar transients likewise have to be considered, because in addition to solar flares, we have to factor in coronal mass ejections (CMEs) and the particles...
Solar Gravity Lens Mission: Refinements and Clarifications
Having just discussed whether humans – as opposed to their machines – will one day make interstellar journeys, it’s a good time to ask where we could get today with near-term technologies. In other words, assuming reasonable progress in the next few decades, what would be the most likely outcome of a sustained effort to push our instruments into deep space? My assumption is that fusion engines will one day be available for spacecraft, but probably not soon, and antimatter, that quixotic ultimate power source for interstellar flight, is a long way from being harnessed for propulsion. We’re left with conventional rocket propulsion with gravity assists, and sail technologies, which not coincidentally describes the two large interstellar missions currently being considered for the heliophysics decadal study. Both JHU/APL’s Interstellar Probe mission and JPL’s SGLF (Solar Gravity Lens Focal) mission aim at reaching well beyond our current distance holders, the now struggling Voyagers. The...
Reaching Proxima b: The Beauty of the Swarm
NIAC’s award of a Phase I grant to study a ‘swarm’ mission to Proxima Centauri naturally ties to Breakthrough Starshot, which continues its interstellar labors, though largely out of the public eye. The award adds a further research channel for Breakthrough’s ideas, and a helpful one at that, for the NASA Innovative Advanced Concepts program supports early stage technologies through three levels of funding, so there is a path for taking these swarm ideas further. An initial paper on swarm strategies was indeed funded by Breakthrough and developed through Space Initiatives and the UK-based Initiative for Interstellar Studies. Centauri Dreams readers are by now familiar with my enthusiasm for swarm concepts, and not just for interstellar purposes. Indeed, as we develop the technologies to send tiny spacecraft in their thousands to remote targets, we’ll be testing the idea out first through computer simulation but then through missions within our own Solar System. Marshall Eubanks, the...
Mars Agriculture – Knowledge Gaps for Regolith Preparation
Let’s break for a moment with interstellar issues to finish up a story I first covered at the beginning of the year. In 2022, members of the Interstellar Research Group led by Doug Loss began exploring the biological side of establishing a human presence on Mars. By ‘biological,’ what the team was looking at was how to create soil as opposed to regolith, soil with the microbial components needed to produce crops for human consumption on Mars. Alex Tolley wrote the idea up in MaRMIE: The Martian Regolith Microbiome Inoculation Experiment. Today’s post is the finalized document that has grown out of this effort, an attempt to foster further research by offering a framework for experiment. While the IRG lacks the means of executing these experiments itself, it offers this paper as a contribution to planetary studies to connect with those who can. by Alex Tolley and Doug Loss* * Contact: Doug Loss at douglas.loss@irg.space Abstract The proposed designs for the settlement of Mars include...
The Realities of Interstellar Hibernation
Larry Niven played around with an interesting form of suspended animation in his 1966 Ballantine title World of Ptavvs. While the usual science fictional imagining is of a crew in some sort of cryogenic deep freeze, Niven went all out and envisioned a means of suspending time itself. It’s an ingenious concept based on an earlier short story in Worlds of Tomorrow, one that so aggressively pushes the physics that the more subtle delights of characterization and perspective come almost as afterthoughts. Niven fans like myself will recognize it as taking part in his ‘Known Space’ universe. In the absence of time manipulation, let’s plumb more modest depths, though these can be tantalizing in their implications. In the last post, Don Wilkins described new work out of Washington University on inducing states of torpor – life processes slowed along with temperature – in laboratory experiments involving rodents. The spectrum from torpor to suspended animation has intervals that may suit our...
Infrastructure and the Interstellar Probe
The question of infrastructure haunts the quest to achieve interstellar flight. I’ve always believed that we will develop deep space capabilities not only for research and commerce but also as a means of defense, ensuring that we will be able to change the trajectories of potentially dangerous objects. But consider the recent Breakthrough Starshot discussion. There I noted that we might balance the images we could receive through Starshot’s sails with those we could produce through telescopes at the Sun’s gravitational focus. Without the infrastructure issue, it would be a simple thing to go with JPL’s Solar Gravitational Lens concept since the target, somewhere around 600 AU, is so much closer, and could produce perhaps even better imagery. But let’s consider Starshot’s huge photon engine in the Atacama desert not as a one-shot enabler for Proxima Centauri, but as a practical tool that, once built, will allow all kinds of fast missions within the Solar System. The financial outlay...
Reflections on Breakthrough Starshot
If we’re going to get to the stars, the path along the way has to go through an effort like Breakthrough Starshot. This is not to say that Breakthrough will achieve an interstellar mission, though its aspirational goal of reaching a nearby star like Proxima Centauri with a flight time of 20 years is one that takes the breath away. But aspirations are just that, and the point is, we need them no matter how far-fetched they seem to drive our ambition, sharpen our perspective and widen our analysis. Whether we achieve them in their initial formulation cannot be known until we try. So let’s talk for a minute about what Starshot is and isn’t. It is not an attempt to use existing technologies to begin building a starship today. Yes, metal is being bent, but in laboratory experiments and simulated environments. No, rather than a construction project, Starshot is about clarifying where we are now, and projecting where we can expect to be within a reasonable time frame. In its early stages,...
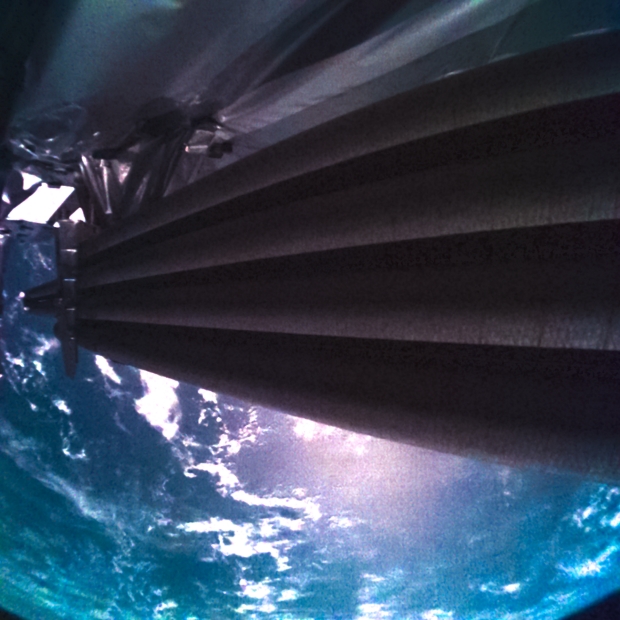
Working the Problem: Deep Space Repair
RIME (Radar for Icy Moons Exploration) is the first instrument ever deployed to the outer Solar System that can make direct measurements of conditions below the surface of an object. That makes it precisely tailored for Europa as well as Ganymede and Callisto, two other Galilean moons that also seem to have an internal ocean. Consider it a radar ‘sounder’ that can penetrate up to 9 kilometers below surface ice. RIME is a major part of why JUICE is going to the moons of Jupiter. Consider it problematic as well, at least for the moment, while controllers working the JUICE mission try to solve an unexpected deployment issue. The 16-meter long antenna shows movement, but continues to have trouble in becoming released from its mounting bracket. The antenna is currently about a third of its full intended length, according to ESA, partially extended but still stowed away. Image: Shortly after launch on 14 April, ESA’s Jupiter Icy Moons Explorer, JUICE, captured this image with its JUICE...
Go JUICE
Take a look at our missions to Jupiter in context. The image below shows the history back to 1973, with the launch of Pioneer 10, and of course, the Voyager encounters. We also have the flybys by Ulysses, Cassini and New Horizons, each designed for other destinations, for Jupiter offers that highly useful gravitational assist to help us get places fast. JUICE (Jupiter Icy Moons Explorer) joins the orbiter side of the image tomorrow, with launch aboard an Ariane 5 from Kourou (French Guiana) scheduled for 1215 UTC (0815 EDT) on Thursday. You can follow the launch live here or here. The first gravitational maneuver will be in August of next year with a Lunar-Earth flyby, followed by Venus in 2025 and then two more Earth flybys (2026 and 2029) before arrival at Jupiter in July of 2031. I’ve written a good deal about both Europa Clipper and JUICE in these pages and won’t go back to repeat the details, but we can expect 35 icy moon flybys past Europa, Ganymede and Callisto before...
A Mission Architecture for the Solar Gravity Lens
Over the past several years we’ve looked at two missions that are being designed to go beyond the heliosphere, much farther than the two Voyagers that are our only operational spacecraft in what we can call the Local Interstellar Medium. Actually, we can be more precise. That part of the Local Interstellar Medium where the Voyagers operate is referred to as the Very Local Interstellar Medium, the region where the LISM is directly affected by the presence of the heliosphere. The Interstellar Probe design from Johns Hopkins Applied Physics Laboratory and the Jet Propulsion Laboratory’s Solar Gravity Lens (SGL) mission would pass through both regions as they conduct their science operations. Both probes have ultimate targets beyond the VLISM, with Interstellar Probe capable of looking back at the heliosphere as a whole and reaching distances are far as 1000 AU still operational and returning data to Earth. The SGL mission begins its primary science mission at the Sun’s gravitational...
Sunvoyager’s Pedigree: On the Growth of Interstellar Ideas
Kelvin Long’s new paper on the mission concept called Sunvoyager would deploy inertial confinement fusion, described in the last post, to drive a spacecraft to 1000 AU in less than four years. The number pulsates with possibilities: A craft like this would move at 325 AU per year, or roughly 1500 kilometers per second, ninety times the velocity of Voyager 1. This kind of capability, which Long thinks we may achieve late in this century, would open up all kinds of fast science missions to the outer planets, the Kuiper Belt, and even the inner Oort Cloud. And the conquest of inertial confinement methods would open the prospect for later, still faster missions to nearby stars. Sunvoyager draws on the heritage of the Daedalus starship, that daring design conceived by British Interplanetary Society members in the 1970s, but as we saw last time, inertial confinement fusion (ICF) was likewise examined in a concept called Vista, and one of the pleasures of this kind of research for a...
SunVoyager: A Fast Fusion Mission Beyond the Heliosphere
1000 AU makes a fine target for our next push past the heliosphere, keeping in mind that good science is to be had all along the way. Thus if we took 100 years to get to 1000 AU (and at Voyager speeds it would be a lot longer than that), we would still be gathering solid data about the Kuiper Belt, the heliosphere itself and its interactions with the interstellar medium, the nature and disposition of interstellar dust, and the plasma environment any future interstellar craft will have to pass through. We don’t have to get there fast to produce useful results, in other words, but it sure would help. The Thousand Astronomical Unit mission (TAU) was examined by NASA in the 1980s using nuclear electric propulsion technologies, one specification being the need to reach the target distance within 50 years. It’s interesting to me – and Kelvin Long discusses this in a new paper we’ll examine in the next few posts – that a large part of the science case for TAU was stellar parallax, for...
Interstellar Communications: The Pointing Problem
Some topics just take off on their own. Several days ago, I began working on a piece about Europa Clipper's latest news, the installation of the reaction wheels that orient the craft for data return to Earth and science studies at target. But data return is one thing for spacecraft working at radio frequencies within the Solar System, and another for much more distant craft, perhaps in interstellar space, using laser methods. So spacecraft orientation in the Solar System triggered my recent interest in the problem of laser pointing beyond the heliosphere, which is acute for long-haul spacecraft like Interstellar Probe, a concept we've recently examined. Because unlike radio methods, laser communications involve an extremely tight, focused beam. Get far enough from the Sun and that beam will have to be exquisitely precise in its placement. So let's take a quick look at Europa Clipper's methods for orienting itself in space, and Voyager's as well, and then move on to how Interstellar...
Interstellar Probe: Prospects for ESA Technologies
The Interstellar Probe concept being developed at Johns Hopkins Applied Physics Laboratory is not alone in the panoply of interstellar studies. We've examined the JHU/APL effort in a series of articles, the most recent being NASA Interstellar Probe: Overview and Prospects. But we should keep in mind that a number of white papers have been submitted to the European Space Agency in response to the effort known as Cosmic Vision and Voyage 2050. One of these, called STELLA, has been put forward to highlight a potential European contribution to the NASA probe beyond the heliosphere. Image: A broad theme of overlapping waves of discovery informs ESA's Cosmic Vision and Voyage 2050 report, here symbolized by icy moons of a gas giant, an temperate exoplanet and the interstellar medium itself, with all it can teach us about galactic evolution. Among the projects discussed in the report is NASA's Interstellar Probe concept. Credit: ESA. Remember that Interstellar Probe (which needs a catchier...
Solar Gravitational Lens: Sailcraft and In-Flight Assembly
The last time we looked at the Jet Propulsion Laboratory’s ongoing efforts toward designing a mission to the Sun’s gravitational lens region beyond 550 AU, I focused on how such a mission would construct the image of a distant exoplanet. Gravitational lensing takes advantage of the Sun’s mass, which as Einstein told us distorts spacetime. A spacecraft placed on the other side of the Sun from the target exoplanetary system would take advantage of this, constructing a high resolution image of unprecedented detail. It’s hard to think of anything short of a true interstellar mission that could produce more data about a nearby exoplanet. In that earlier post, I focused on one part of the JPL work, as the team under the direction of Slava Turyshev had produced a paper updating the modeling of the solar corona. The new numerical simulations led to a powerful result. Remember that the corona is an issue because the light we are studying is being bent around the Sun, and we are in danger of...
Good News for a Gravitational Focus Mission
We’ve talked about the ongoing work at the Jet Propulsion Society on the Sun’s gravitational focus at some length, most recently in JPL Work on a Gravitational Lensing Mission, where I looked at Slava Turyshev and team’s Phase II report to the NASA Innovative Advanced Concepts office. The team is now deep into the work on their Phase III NIAC study, with a new paper available in preprint form. Dr. Turyshev tells me it can be considered a summary as well as an extension of previous results, and today I want to look at the significance of one aspect of this extension. There are numerous reasons for getting a spacecraft to the distance needed to exploit the Sun’s gravitational lens – where the mass of our star bends the light of objects behind it to produce a lens with extraordinary properties. The paper, titled “Resolved Imaging of Exoplanets with the Solar Gravitational Lens,” notes that at optical or near-optical wavelengths, the amplification of light is on the order of ~ 2 X 1011,...