by Paul Gilster | Jan 11, 2022 | Sail Concepts |
I’ve learned that you can’t assume anything when giving a public talk about the challenge of interstellar flight. For a lot of people, the kind of distances we’re talking about are unknown. I always start with the kind of distances we’ve reached with spacecraft thus far, which is measured in the hundreds of AUs. With Voyager 1 now almost 156 AU out, I can get a rise out of the audience by showing a slide of the Earth at 1 AU, and I can mention a speed: 17.1 kilometers per second. We can then come around to Proxima Centauri at 260,000 AU. A sense of scale begins to emerge.
But what about propulsion? I’ve been thinking about this in relation to a fundamental gap in our aspirations, moving from today’s rocketry to what may become tomorrow’s relativistic technologies. One thing to get across to an audience is just how little certain things have changed. It was exhilarating, for example, to watch the Arianne booster carry the James Webb Space Telescope aloft, but we’re still using chemical (and solid state) engines that carry steep limitations. Rockets using fission and fusion engines could ramp up performance, with fusion in particular being attractive if we can master it. But finding ways to leave the fuel behind may be the most attractive option of all.
I was corresponding with Philip Lubin (UC-Santa Barbara) about this in relation to a new paper we’ll be looking at over the next few days. Dr. Lubin makes a strong point on where rocketry has taken us. Let me quote him from a recent email:
…when you look at space propulsion over the past 80 years, we are still using the same rocket design as the V2 only larger But NOT faster. Hence in 80 years we have made incredible strides in exploring our solar system and the universe but our propulsion system is like that of internal combustion engine cars. No real change. Just bigger cars. So for space exploration to date – “just bigger rockets” but “not faster rockets”. [SpaceX’s] Starship is incredible and I love what it will do for humanity but it is fundamentally a large V2 using LOX and CH4 instead of LOX and Alcohol.
The point is that we have to do a lot better if we’re going to talk about practical missions to the stars. Interstellar flight is feasible today if we accept mission durations measured in thousands of years (well over 70,000 years at Voyager 1 speeds to travel the distance to Proxima Centauri). But taking instrumented probes, much less ships with human crews, to the nearest star demands a completely different approach, one that Lubin and team have been exploring at UC-SB. Beamed or ‘directed energy’ systems may do the trick one day if we can master both the technology and the economics.
Let’s ponder what we’re trying to do. Lubin likes to show the diagram below, which brings out some fundamental issues about how we bring things up to speed. On the one hand we have chemical propulsion, which as the figure hardly needs to note, is not remotely relativistic. At the high end, we have the aspirational goal of highly relativistic acceleration enabled by directed energy – a powerful beam pushing a sail.
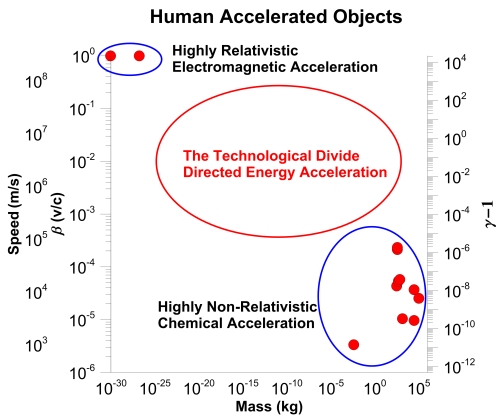
Image: This is Figure 1 from “The Economics of Interstellar Flight,” by Philip Lubin and colleague Alexander Cohen (citation below). Caption: Speed and fractional speed of light achieved by human accelerated objects vs. mass of object from sub-atomic to large macroscopic objects. Right side y-axis shows ? ? 1 where ? is the relativistic “gamma factor.” ? ? 1 times the rest mass energy is the kinetic energy of the object.
Thinking again of how I might get this across to an audience, I fall back on the energies involved, for as Lubin and Cohen’s paper explains, the energy available in chemical bonds is simply not sufficient for our purposes. It is mind-boggling to follow this through, as the authors do. Take the entire mass of the universe and turn it into chemical propellant. Your goal is to accelerate a single proton with this unimaginable rocket. The final speed you achieve is in the range of 300 to 600 kilometers per second.
That’s fast by Voyager standards, of course, but it’s also just a fraction of light speed (let’s give this a little play and say you might get as high as 0.3 percent), and the payload is no more than a single proton! We need energy levels a billion times that of chemical reactions. We do know how to accelerate elementary particles to relativistic velocities, but as the universe-sized ‘rocket’ analogy makes clear, we can’t dream of doing this through chemical energy. Particle accelerators reach these velocities with electromagnetic means, but we can’t yet do it beyond the particle level.
Directed energy offers us a way forward but only if we can master the trends in photonics and electronics that can empower this new kind of propulsion in realistic missions. In their new paper, to be published in a special issue of Acta Astronautica, Lubin and Cohen are exploring how we might leverage the power of growing economies and potentially exponential growth in enough key areas to make directed energy work as an economically viable, incrementally growing capability.
Beaming energy to sails should be familiar territory for Centauri Dreams readers. For the past eighteen years, we’ve been looking at solar sails and sails pushed by microwave or laser, concepts that take us back to the mid-20th Century. The contribution of Robert Forward to the idea of sail propulsion was enormous, particularly in spreading the notion within the space community, but sails have been championed by numerous scientists and science fiction authors for decades. Jim Benford, who along with brother Greg performed the first laboratory work on beamed sails, offers a helpful Photon Beam Propulsion Timeline, available in these pages.
In the Lubin and Cohen paper, the authors make the case that two fundamental types of mission spaces exist for beamed energy. What they call Direct Drive Mode (DDM) uses a highly reflective sail that receives energy via momentum transfer. This is the fundamental mechanism for achieving relativistic flight. Some of Bob Forward’s mission concepts could make an interstellar crossing within the lifetime of human crews. In fact, he even developed braking methods using segmented sails that could decelerate at destination for exploration at the target star and eventual return.
Lubin and Cohen also see an Indirect Drive Mode (IDM), which relies on beamed energy to power up an onboard ion engine that then provides the thrust. My friend Al Jackson, working with Daniel Whitmire, did an early analysis of such a system (see Rocketry on a Beam of Light), The difference is sharp: A system like this carries fuel onboard, unlike its Direct Drive Mode cousin, and thus has limits that make it best suited to work within the Solar System. While ruling out high mass missions to the stars, this mode offers huge advantages for reaching deep into the system, carrying high mass payloads to the outer planets and beyond. From the paper:
…for the same mission thrust desired, an IDM approach uses much lower power BUT achieves much lower final speed. For solar system missions with high mass, the final speeds are typically of order 100 km/s and hence an IDM approach is generally economically preferred. Another way to think of this is that a system designed for a low mass relativistic mission can also be used in an IDM approach for a high mass, low speed mission.
We shouldn’t play down IDM because it isn’t suited for interstellar missions. Fast missions to Mars are a powerful early incentive, while projecting power to spacecraft and eventual human outposts deeper in the Solar System is a major step forward. Beamed propulsion is not a case of a specific technology for a single deep space mission, but rather a series of developing systems that advance our reach. The fact that such systems can play a role in planetary defense is a not inconsiderable benefit.
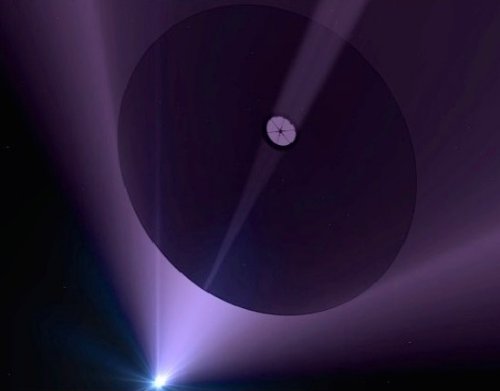
Image: Beamed propulsion leaves propellant behind, a key advantage. It could provide a path for missions to the nearest stars. Credit: Adrian Mann.
If we’re going to analyze how we go from here, where we’re at the level of lab experiments, to there, with functioning directed energy missions, we have to examine these trends in terms of their likely staging points. What I mean is that we’re looking not at a single breakthrough that we immediately turn into a mission, but a series of incremental steps that ride the economic wave that can drive down costs. Each incremental step offers scientific payoff as our technological prowess develops.
Getting to interstellar flight demands patience. In economic terms, we’re dealing with moving targets, making the assessment at each stage complicated. Think of photovoltaic arrays of the kind we use to feed power to our spacecraft. As Lubin and Cohen point out, until recently the cost of solar panels was the dominant economic fact about implementing this technology. Today, this is no longer true. Now it’s background factors – installation, wiring, etc. – that dominate the cost. We’ll get into this more in the next post, but the point is that when looking at a long-term outcome, we have a number of changing factors that must be considered.
Some parts of a directed energy system show exponential growth, such as photonics and electronics. And some do not. The cost of metals, concrete and glass move at anything but exponential rates. What “The Economics of Interstellar Flight” considers is developing a cost model that minimizes the cost for a specific outcome.
To do this, the authors have to consider the system parameters, such things as the power array that will feed the spacecraft, its diameter, the wavelength in use. And you can see the complication: When some key technologies are growing at exponential rates, time becomes a major issue. A longer wait means lower costs, while the cost of labor, land and launch may well increase with time. We can also see a ‘knowledge cost’: Wait time delays knowledge acquisition. As the authors note in relation to lasers:
The other complication is that many system parameters are interconnected and there is the severe issue that we do not currently have the capacity to produce the required laser power levels we will need and hence industrial capacity will have to catch up, but we do not want to be the sole customer. Hence, finding technologies that are driven by other sectors or adopting technologies produced in mass quantity for other sectors may be required to get to the desired economic price point.
System costs, in other words, are dynamic, given that some technologies are seeing exponential growth and others are not, making a calculation of what the authors call ‘time of entry’ for any given space milestone a challenging goal. I want to carry this discussion of how the burgeoning electronics and photonics industries – driven by power trends in consumer spending – factor into our space ambitions into the next post. We’ll look at how dreams of Centauri may eventually be achieved through a series of steps that demand a long-term, deliberate approach relying on economic growth.
The paper is Lubin & Cohen, “The Economics of Interstellar Flight,” to be published in Acta Astronautica (preprint).
by Paul Gilster | Nov 19, 2021 | Sail Concepts |
Can you imagine the science we could do if we had the capability of sending a probe to Jupiter with travel time of less than a month? How about Neptune in 18 weeks? Alex Tolley has been running the numbers on a concept called Wind Rider, which derives from the plasma magnet sail he has analyzed in these pages before (see, for example, The Plasma Magnet Drive: A Simple, Cheap Drive for the Solar System and Beyond). The numbers are dramatic, but only testing in space will tell us whether they are achievable, and whether the highly variable solar wind can be stably harnessed to drive the craft. A long-time contributor to Centauri Dreams, Alex is co-author (with Brian McConnell) of A Design for a Reusable Water-Based Spacecraft Known as the Spacecoach (Springer, 2016), focusing on a new technology for Solar System expansion.
by Alex Tolley
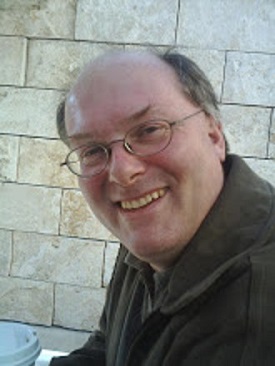
In 2017 I outlined a proposed magnetic sail propulsion system called the Plasma Magnet that was presented by Jeff Greason at an interstellar conference [6]. It caught my attention because of its simplicity and potential high performance compared to other propulsion approaches. For example, the Breakthrough Starshot beamed sail required hugely powerful and expensive phased-array lasers to propel a sail into interstellar space. By contrast, the Plasma Magnet [PM] required relatively little energy and yet was capable of propelling a much larger mass at a velocity exceeding any current propulsion system, including advanced solar sails.
The Plasma Magnet was proposed by Slough [5] and involved an arrangement of coils to co-opt the solar wind ions to induce a very large magnetosphere that is propelled by the solar wind. Unlike earlier proposals for magnetic sails that required a large electric coil kilometers in diameter to create the magnetic field, the induction of the solar wind ions to create the field meant that the structure was both low mass and that the size of the resulting magnetic field increased as the surrounding particle density declined. This allowed for a constant acceleration as the PM was propelled away from the sun, very different from solar sails and even magsails with fixed collecting areas.
The PM concept has been developed further with a much sexier name: the Wind Rider, and missions to use this updated magsail vehicle are being defined.
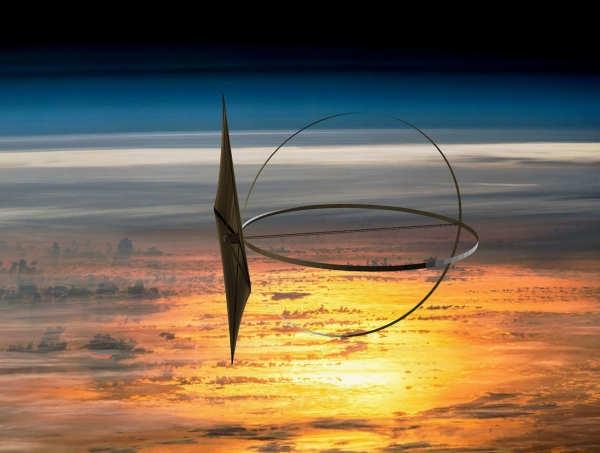
Wind Rider was presented at the 2021 Division of Planetary Sciences (DPS) meeting by the team led by Brent Freeze, showing their concept of the design for a Jupiter mission they called JOVE. The December meeting of the American Geophysical Union was the venue for a different Wind Rider concept mission to the SGL, called Pathfinder.
The main upgrade from the earlier PM to the Wind Rider is the substitution of superconducting coils. This allows the craft to maintain the magnetic field without requiring constant power to maintain the electric current, reducing the required power source. Because the superconducting coils would quickly heat up in the inner system and lose their superconductivity, a gold foil reflective sun shield is deployed to shield the coils from the sun’s radiation. This is shown in the image above with the shield facing the sun to keep the coils in shadow. The shield is also expected to do double duty as a radio antenna, reducing the net parasitic mass on the vehicle.
The performance of the Wind Rider is very impressive. Calculations show that it will accelerate very rapidly and reach the velocity of the solar wind, about 400 km/s. This has implications for the flight trajectory of the vehicle and the mission time.
The first mission proposal is a flyby of Jupiter – Jupiter Observing Velocity Experiment (JOVE) – much like the New Horizons mission did at Pluto.
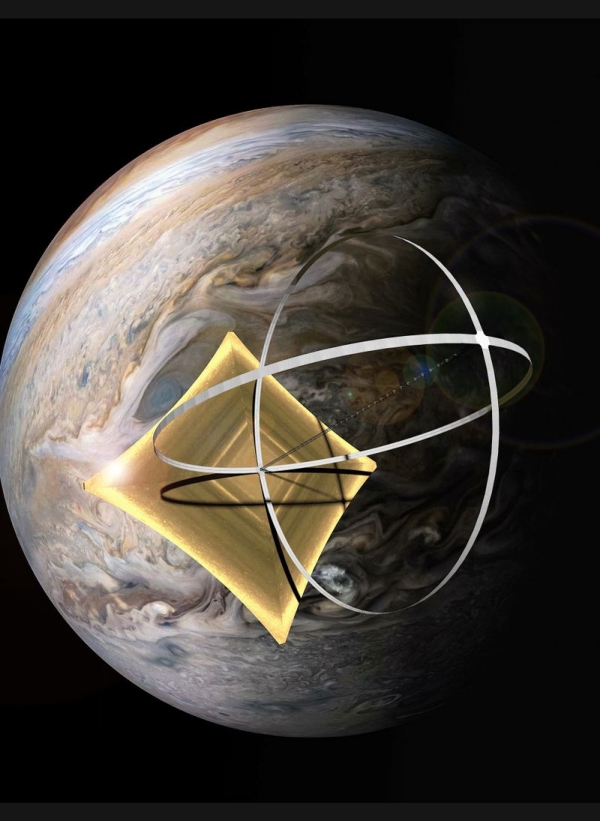
Figure 1. The Wind Rider on a flyby of Jupiter. The solar panels are hidden behind the sun shield facing the sun. The 16U CubeSat chassis is at the intersection of the 2 coils and sun shield.
The JOVE mission proposal is for an instrumented flyby of Jupiter [2]. The chassis is a 16U CubeSat. The scientific instrument payload is primarily to measure data on the magnetic field and ion density around Jupiter. The sail is powered by 4 solar panels that also double as struts to support the sun shield and generate about 1300 W at 1 AU and fall to about 50W at Jupiter.
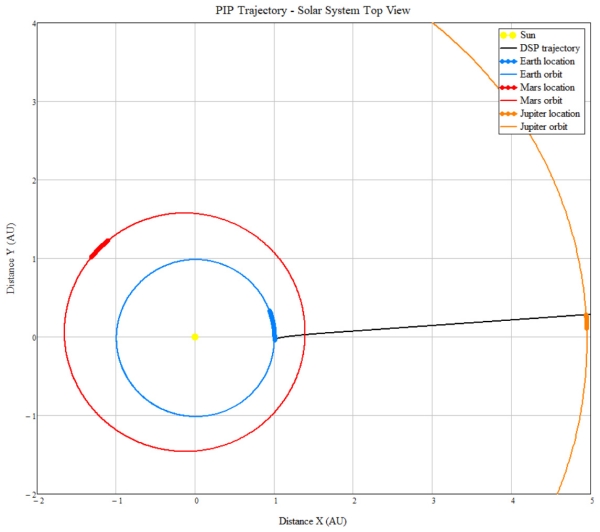
Figure 2. Trajectory of the Wind Rider from Earth to Jupiter
The flight trajectory is effectively a beeline directly to Jupiter, starting the flight almost at opposition. No gravity assists from Earth or Venus are required, nor a long arcing trajectory to intercept Jupiter. Figure 2 shows the trajectory, which is almost a straight-line course with the average velocity close to that of the solar wind.
Although the mission is planned as a flyby, a future mission could allow for orbital insertion if the craft approaches Jupiter’s rotating magnetosphere to maximize the impinging field velocity. Although not mentioned by the authors, it should be noted that Slough has also proposed using a PM as an aerobraking shield that decelerates the craft as it creates a plasma in the upper atmosphere of planets.
How does the performance of the Wind Rider compare to other comparable missions?
The JUNO space probe to Jupiter had a maximum velocity of about 73 km/s as Jupiter’s gravity accelerated the craft towards the planet. The required gravity assists and long flight path, about 63 AU or over 9 billion km, mean that its average velocity was about 60 km/s. This is not the fairest comparison as the JUNO probe had to attain orbital insertion at Jupiter.
A fairer comparison is the fastest probe we have flown – the New Horizons mission to Pluto — which reached 45 km/s as it left Earth but slowed to 14 km/s as it flew by Pluto. New Horizons took 1 year to reach Jupiter to get a gravity assist for its 9 year mission to Pluto, and therefore a maximum average velocity of 19 km/s between Earth and Jupiter.
Wind Rider can reach Jupiter in less than a month. Figure 2 shows the almost straight-line trajectory to Jupiter. Launched just before opposition, Wind Rider reaches Jupiter in just over 3 weeks. Because opposition happens annually, a new mission could be launched every year.
As the Wind Rider quickly reaches its terminal velocity at the same velocity as the solar wind, it can reach the outer planets with comparably short times with the same trajectory and annual launch windows.
The Wind Rider can fly by Saturn in just 6 weeks, and Neptune in 18 weeks. Compare that to the Voyager 2 probe launched in 1977 that took 4 years and 12 years to fly by the same planets respectively. Pluto could be reached by Wind Rider in just 6 months.
Because of its high terminal velocity that does not reduce during its mission, the Wind Rider is also ideally suited for precursor interstellar missions.
The second proposed mission is called Pathfinder [1], proposed to ultimately reach the solar gravity focal line around 550 AU from the sun. Flight time is less than 7 years, making this a viable project for a science and engineering team and not a multi-generation one based on existing rocket propulsion technology. As the flight trajectory is a straight line, this makes the craft well suited to follow the focal line while imaging a target star or exoplanet using the sun’s diameter as a large aperture telescope to increase the resolving power.
As the Wind Rider reaches the solar wind velocity, it may even be able to ride the gusts of higher solar wind velocities, perhaps reaching closer to 550 km/s.
While solar sails have been considered the more likely means to reach high velocities, especially when making sun-diver maneuvers, even advanced sails with proposed areal densities well below anything available today would reach solar system escape velocities in the range of 80-120 km/s [3]. If the Wind Rider can indeed reach the velocity of the solar wind, it would prove a far faster vehicle than any solar sail being planned, and would not need a boost from large laser arrays, nor risky sun-diver maneuvers.
I would inject some caution at this point regarding the performance. The performance is based entirely on theoretical work and a small scale laboratory experiment. What is needed is a prototype launched into cis-lunar space to test the performace on actual hardware and confirm the capability of the technology to operate as theorized.
It should also be noted that despite its theoretical high performance, there is a potential issue with propelling a probe with a magnetic sail. Compared to a solar sail or a vehicle with reaction thrusters, the Wind Rider as described so far has no crosswind capability. It just runs in front of the solar wind like a dandelion seed in the wind. This means that it would have to be aimed very accurately at its target, and subject to the vagaries of the strength of the solar wind that is far less stable than the sun’s photon emissions. Like the dandelion, if the Wind Rider was very inexpensive, many could be launched in the expectation that at least one would successfully reach its target.
However, there is a possibility that some crosswind capability is possible. This is based on modelling by Nishida [4]. This paper was recommended by Dr. Freeze [7].
The study modeled the effect of the angle of attack of the magnetic field of a coil against the solar wind. The coil in this case would represent the induced circular movement of the solar wind induced by the primary Wind Rider/PM coils.
Theoretically, the angle of attack has an impact on the total force pushing past the magnetic field.
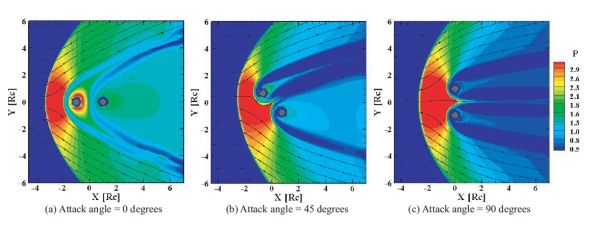
Figure 3 shows the pressure and on the field as the coil is rotated from 0 through 45 and 90 degrees to the solar wind.
The force experienced is maximal at 90 degrees. This is shown visually in figure 3 and graphically in figure 4.
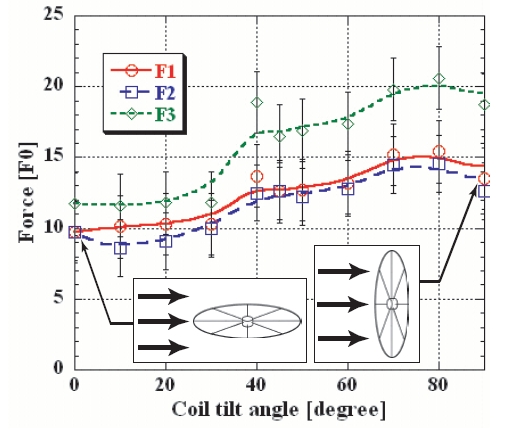
Figure 4. Force on the coil effected by angle of attack. A near 90 degrees angle of attack increases the force about 50%.
The angle of attack also induces a change in the thrust vector experienced by the coil, which would act as a crosswind maneuvering capability, allowing for trajectory adjustments as well as a longer launch window for the Wind Rider.
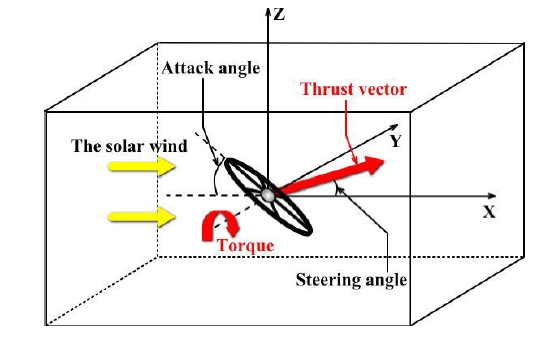
Figure 5. The angle of attack affects the thrust vector. But note the countervailing torque on the coil.
If the coil can maintain an angle of attack with respect to teh solar wind, then the Wind Rider can steer across the solar wind to some extent.
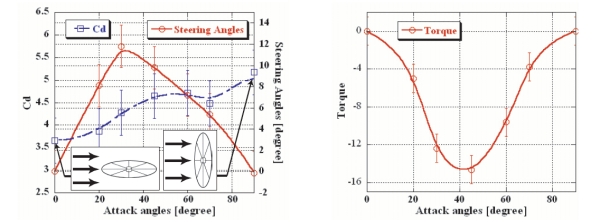
Figure 6. (left) Angle of attack, and steering angle. (right) angle of attack and the torque on the coil.
Figure 6 shows that the craft could steer up to 12 degrees away from the solar wind direction. However, maintaining that angle of attack requires a constant force to oppose the torque restoring the angle of attack to zero or 90 degrees. The coil therefore acts like a weather vane, always trying to align itself with the solar wind. To maintain the angle of attack would be difficult. Reaction wheels like those on the Kepler telescope could only act in a transient manner. Another possibility suggested is to move the center of gravity of the craft in some way. Adding booms with coils might be another solution, albeit by adding mass and complexity, undesirable for this first generation probe. Jeff Greason has an upcoming paper to be published in 2022 on theoretical navigation with possible ranges of steering capability.
In summary, the Wind Rider is an upgraded version of the Plasma Magnet propulsion concept, now applied to a reference design for 2 missions, a fast flyby of Jupiter, and an interstellar precursor mission that could reach the solar gravity lens focus. The performance of the design is primarily based on modelling and as yet there is no experimental evidence to support a finite lift/drag ratio for the craft.
Having said that, the propulsion principle and hardware necessary are not expensive, and there seems to be much interest by the AIAA. Maybe this propulsion method can finally be built, flown and evaluated. If it works as advertised, it would open up the solar system to exploration by fast, cheap robotic probes and eventually crewed ships.
References
1. Freeze, B et al Wind Rider Pathfinder Mission to Trappist-1 Solar Gravitational Lens Focal Region in 8 Years (poster at AGU – Dec 13th, 2021). https://agu.confex.com/agu/fm21/meetingapp.cgi/Paper/796237
2. Freeze, B et al Jupiter Observing Velocity Experiment (JOVE), Introduction to Wind Rider Solar Electric Propulsion Demonstrator and Science Objective.
https://baas.aas.org/pub/2021n7i314p05/release/1
3. Vulpetti, Giovanni, et al. (2008) Solar Sails: A Novel Approach to Interplanetary Travel. New York: Springer, 2008.
4. Nishida, Hiroyuki, et al. “Verification of Momentum Transfer Process on Magnetic Sail Using MHD Model.” 41st AIAA/ASME/SAE/ASEE Joint Propulsion Conference & Exhibit, 2005.
https://doi.org/10.2514/6.2005-4463
5. Slough, J. “Plasma Magnet NASA Institute for Advanced Concepts Phase I Final Report.” 2004. http://www.niac.usra.edu/files/studies/final_report/860Slough.pdf. See Figure 2.
6. Tolley, A “The Plasma Magnet Drive: A Simple, Cheap Drive for the Solar System and Beyond” (2017).
https://www.centauri-dreams.org/2017/12/29/the-plasma-magnet-drive-a-simple-cheap-drive-for-the-solar-system-and-beyond/
7. Generous email communications with Dr. Brent Freeze in preparation of this article.
by Paul Gilster | Aug 14, 2020 | Sail Concepts |
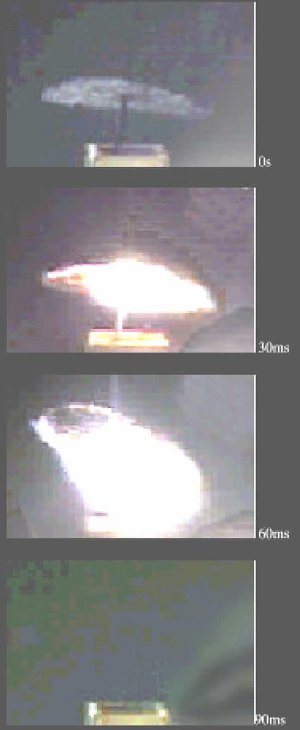
The first laboratory work on pushing a space sail with microwaves was performed by Jim and Greg Benford at the Jet Propulsion Laboratory back in 1999, with the results presented the following year at a European conference. Leik Myrabo (then at Rensselaer Polytechnic Institute) was, at about the same time, performing experiments with lasers at Wright-Patterson Air Force Base in Ohio. When you think about the problems of laboratory work on these matters, consider the fact of gravity, meaning that you are working in a 1 g gravity well with diaphanous materials whose acceleration depends on how hot you can allow them to become.
Advances in materials and in particular in lightweight carbon structures allowed the Benfords’ experiments to succeed, with the help of a 10-kilowatt microwave beam that produced significant acceleration on the test object. But I’m reminded by looking at a new paper on sail technologies using no beam at all that the Benfords also demonstrated something else. Molecules of CO2, hydrocarbons and hydrogen that had been incorporated in the lattice of their material at manufacture emerged under the high temperatures involved. Thus another form of acceleration came into play through the phenomenon called desorption.
Image: Carbon disk sail lifting off of truncated rectangular waveguide under 10 kW microwave power (four frames, 30 ms interval, first at top). Credit: James and Gregory Benford.
Just how useful an effect may desorption turn out to be? It seems to offer an acceleration dividend. Roman Kezerashvili (City University of New York), working with colleagues at Samara National Research University in Russia and the State University of New York at Buffalo, has now analyzed the effect of desorption on an inflated, torus-shaped sail. I had the pleasure of talking with Dr. Kezerashvili in Italy at the Aosta conference in 2009, where he discussed relativistic effects that have to be taken into account in the navigation of a space sail close to the Sun. The current work follows up an earlier paper on desorption he presented in 2016.
We have the prospect of incorporating compounds into a sail that become a kind of propulsive shell that is triggered either by microwave beam or, in the case of a close Solar pass, by the Sun itself. Think of desorption as involving a kind of propulsive ‘paint.’ In terms of beaming, it is necessary to understand that desorption can take place under either microwave or laser beam, but microwaves do not damage sail materials and so heat them far less destructively. The Benfords’ work, as Kezerashvili points out, shows that microwave beaming produces more efficient absorption in the sail’s coating materials, and high specific impulse can result from desorption.
In fact, in the Benford experiments on ultra-light carbon sails, photon pressure can account for no more than 30 percent of the observed acceleration. The rest comes from desorption.
Various materials can undergo desorption at different temperatures — the sail analyzed by Kezerashvili in 2016 uses carbon, whose properties of acceleration are there analyzed. If low mass atoms or molecules can be blown out of the sail lattice at known temperatures, the mission concept Kezerashvili suggested in that 2016 paper can emerge, one in which the sail reaches desorption temperature at a particular point in a close pass around the Sun. In this scenario, no microwave or laser beam is needed:
It is of particular interest to consider an inflatable torus-shaped solar sail as both propellant-less and propellant-based system. It is a propellant-based and a propellant-less system which create thrust by the sun-driven ejection of a flux of particles of non-zero rest mass due to the desorption of coating and solar radiation pressure, while it performs as a propellant-less conventional solar sail after the thermal desorption ends.
As far as I know, this new paper is the first analysis of desorption on an inflatable sail, but inflatable structures have a long history due to their flexibility and low volume at launch. Among the earliest inflatables to be used in space were the Echo balloons launched in the 1960s, but inflatable structures have been developed extensively in the decades since. I’ve written in the past on a hydrogen-inflated sail using a molybdenum reflector that became the basis for an early orbiting radio telescope design, a concept Greg Matloff extended and investigated along with Kezerashvili and Italian physicist Giancarlo Genta for interstellar flight purposes.
This is also the first paper that considers thermal desorption in the context of solar heating alone, which leads to an interesting class of ‘sundiver’ missions with close solar passes. Thus a conventional solar sail coated with desorptive paint is heated by the space environment.
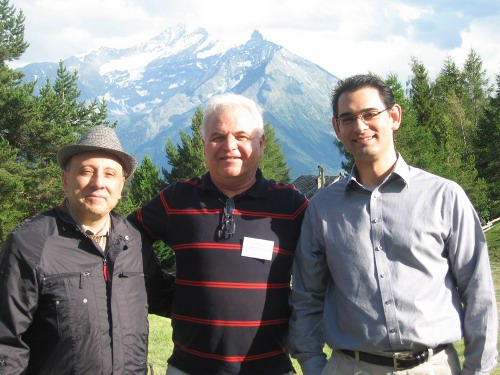
Image: From the Aosta conference of 2009, a fine memory: A snapshot taken in the Italian Alps. Left to right: Giovanni Vulpetti, Roman Kezerashvili, and Justin Vazquez-Poritz.
On deployment matters: Most solar and beamed sails discussed in the literature assume systems of electromagnetic actuation devices, often involving guide rollers and booms. All of this, of course, adds to the mass of the resulting structure as well as its complexity. Deployment through a series of inflatable booms, as assumed in the inflatable sail concept, simplifies an otherwise intricate procedure. The paper also mentions the advances in flexible polymers and high-strength fibers that allow more mass packed into the launch vehicle at lower cost.
So inflatable sails make sense. The new Kezerashvili paper looks at the dynamics of an inflatable sail, conceived as “a thin reflective membrane attached to an inflatable torus-shaped rim.” The round, flat membrane is coated with heat sensitive materials that make the transition from solid state to gas. Pressure introduced into the rim allows sail deployment from the initial stowed configuration, with deployment occurring at the chosen heliocentric distance.
At this point the sail membrane is extended into its final flat shape, with thermal desorption occurring at a specific temperature. For the purposes of these calculations, the authors cite an acceleration time for the torus-shaped solar sail due to thermal desorption of about 1500 seconds, with the mass of the coating material pegged at 1.5 kg and a desorption rate of 1 g/s.
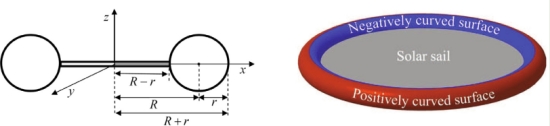
Image: This is Figure 1 from the paper. Credit: Kezerashvili et al.
And here is a figure from one of Dr. Kezerashvili’s presentations on the inflated sail.
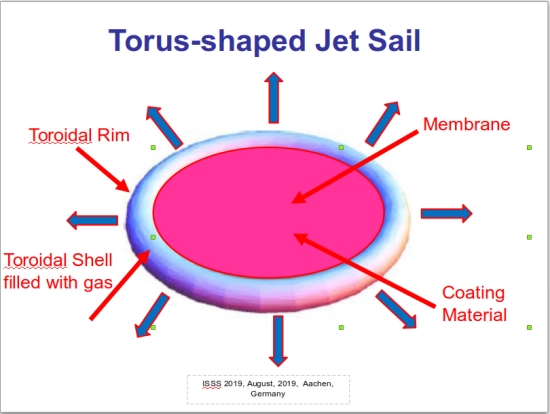
Thus we wind up with two types of acceleration at perihelion, the first being the expected solar radiation pressure, the second being that caused by thermal desorption from the sail itself. The authors assume a beryllium-coated solar sail and analyze the membrane mass of the sail as well as the toroidal rim and coating material, along with the desorption rate, under varying molecular hydrogen gas fills and a temperature range varying with the perihelion approach considered. The different gas fills change the tensile stress on the sail membrane. The paper analyzes the structural strength required in the inflatable torus to support the circular membrane of the sail and considers the deflection of the membrane due to acceleration.
A ‘sundiver’ maneuver is a mission scenario we’ve discussed often in these pages — only this one occurs with an inflatable sail, and it is a mission in which the sail is deployed just as the appropriate temperature is reached. The additional kick provided by desorption produces a substantial boost in performance over a sail driven by solar radiation alone:
The present study reveals that the inflation deployed torus-shaped solar sail accelerated via thermal desorption of coating results in high post-perihelion heliocentric solar sail velocities. With the speed 20-40 AU/year, post-perihelion travel times to the vicinity of Kuiper Belt Objects (KBO) will be less than 1-3 years, while the Sun’s gravity focus at 547 AU can be reached in 13-25 years.
What interested me in particular was the idea of extending the concept into small sails and opting for a ‘fleet’ concept:
The suggested configuration of the torus-shaped solar sail fits the cube-scale size configurations. Recent research reveals that much smaller sails could be incorporated with highly miniaturized chip-scale spacecraft. It is quite possible that a single dedicated interplanetary ”bus” could deploy many cube-scale sails at perihelion. Sequential deployment of a fleet of solar sails could be timed to allow exploration of many small KBOs from a single launch. The natural continuation of this work can be extended in the following directions: i. Detailed research on materials for thermal desorption at temperature suitable for solar sailing; ii. consideration of the Sun as an extended source of radiation; iii. study the influence of solar sail surface oscillations on the motion of a spacecraft performing an interplanetary flight.
Interesting issues arise with a sail like this. The coating on the surface of the sail must be uniform, and in fact one would expect that slight variations will occur that can lead to trajectory deviations during the desorption process. Remember that the time of desorption acceleration is small, around 1500 seconds, in the configuration discussed here. The authors assume there would be a need for periodic corrections in the sail’s tilting angle as these deviations occur. You can also see how neat a fit this work makes with Kezerashvili’s earlier studies on navigation issues deep in a gravity well. An error in relativistic calculations close to the Sun could lead to substantial variations in the final trajectory.
The paper is Kezerashvili et al., “A torus-shaped solar sail accelerated via thermal desorption of coating,” in press at Advances in Space Research (abstract). The original 2016 paper is Ancona and Kezerashvili, “Orbital dynamics of a solar sail accelerated by thermal desorption of coatings,” Proceedings of 67th International Astronautical Congress (IAC 2016), Guadalajara, Mexico, 26-30 September 2016. Paper IAC-16-C1.6.7.32480 (preprint). The comprehensive paper on extrasolar exploration by a solar sail accelerated via thermal desorption of a coating that grew out of this is published in Advances in Space Research 63, pp. 2021-2034 (2019).
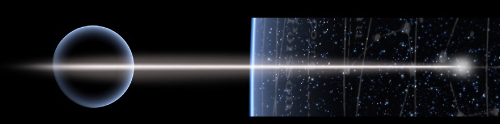
by Paul Gilster | Jul 30, 2020 | Sail Concepts |
I’ve focused on aerographite these past several days because sail materials are a significant determinant of the kind of missions we can fly both in the near-term and beyond. The emergence of a new ‘contender’ to join graphene as a leading candidate for deep space missions is worthy of note. Whether or not this ultra lightweight material produced by teams at the Technical University of Hamburg and the University of Kiel lives up to its promise will depend upon a thorough investigation of its properties as adapted for sails, one which has already begun.
Sail materials matter because we have already begun flying spacecraft with these technologies, so that as we climb the learning curve in terms of design and engineering, we need to be thinking about how to increase performance to allow ambitious missions, and perhaps even audacious ones like Breakthrough Starshot, though the authors of the first paper on aerographite for sails are skeptical about whether the material could withstand the demands of the Starshot laser push.
Aerographite seems to allow strikingly fast missions using solar photons alone — the paper discusses reaching Pluto orbit in less than 5 years, for example — but the authors of this paper are fully aware of the number and complexity of the issues that need to be addressed to make such a thing happen. The idea is to advance the concept, bat the ideas around, learn from laboratory experiment, and try this relatively inexpensive material out in space, starting probably with near-term projects on the International Space Station and going from there.
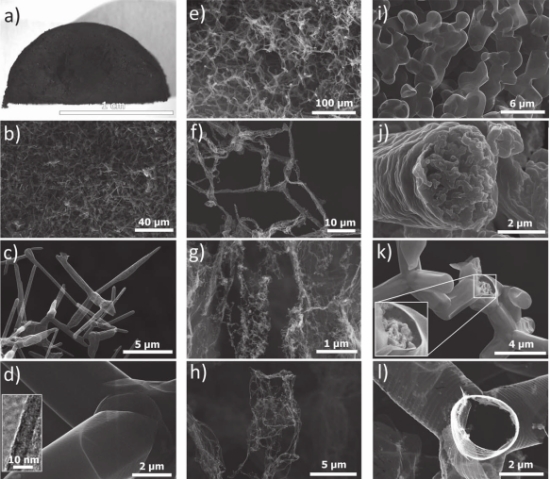
Image: This is Figure 1 from the aerographite discovery paper. Note: TEM = Transmission electron microscopy. The caption: Overview of different Aerographite morphologies by controlled derivations of synthesis. a) Photograph of macroscopic Aerographite. b–d) 3D interconnected structure of closed-shell graphitic Aerographite in different magnifications and TEM inset of wall. e–h) Hierarchical hollow framework configuration of Aerographite in different magnifications. i–l) Other variants of Aerographite. i) Aerographite network in low aspect bubble-like configuration. j-k) Aerographite with nano porous graphite filling. l) Hollow corrugated pipe design of Aerographite surface by detailed adoption of template shape. Credit: Mecklenburg et al.
Among the issues I didn’t have time to discuss yesterday is the sail’s absorption of photons from a high-energy beam, like Starshot’s projected ground-based array. An aerographite sail that absorbs solar photons may have trouble under an intense beam, considering that the material melts at 400 C — remember, we are talking about a black sail that works through absorbance rather than reflectivity. 400 C is a figure that the Hamburg team measured under atmospheric conditions — consider it a combustion temperature, as Alex Tolley reminds me, rather than a melting point — so we would still need to learn about the situation in space. This would be a matter of simple experiment but a crucial issue to resolve if we’re interested in beamed sails.
A larger problem emerges with the fact that aerographite is an electrical as well as a heat conductor. That means an aerographite sail will accumulate charge from solar UV radiation or possibly the solar wind, as the paper is quick to note. Launching an aerographite sail from low Earth orbit could result in deflection of the sail’s trajectory by Lorentz forces induced by the Earth’s magnetic field. Interplanetary and interstellar magnetic fields pose a challenge depending on the mission.
Thus we have a navigation issue to investigate, one that would likely need to be resolved, says lead author René Heller (Max Planck Institute for Solar System Research, Göttingen) by an active, autonomous on-board computer “…and some form of photon “wings” or “rudders” to trim the sail.” The effect of charge upon aerographite can be viewed in a video: https://www.youtube.com/watch?v=Oh8skH1oQDE&feature=emb_title.
Heller said in an email discussion that before we start talking about interstellar missions, we need to figure out how to move an aerographite sail in predictable ways:
At this point, we are introducing the basic equations and some sample trajectories to show that interstellar escape is possible in principle. But steering is very complicated. There are so many unknowns that would affect a sail trajectory such as interplanetary magnetic fields (leads to deflection if the sail gets electrically charged, e.g. by cosmic particle hits), the solar wind, interstellar magnetic fields, limited knowledge of the actual position and velocity (“proper motion”) of the target star etc. that I can’t see at this point how one could passively steer an aerographite hollow sphere or cone or parachute web – whatever – to a star at 4 light years. That’s why we entitled our paper an “interstellar precursor”. Even aiming at Mars would be hard with a passive sail, which is why we talk about reaching the orbit of Mars and the orbit of Pluto rather than Mars and Pluto themselves.
Structural reinforcement may turn out to be necessary given the material’s relatively low tensile strength. All of these matters need investigation, but the beauty of aerographite is that it is available for demonstrator work near Earth, as co-author Pierre Kervella adds:
…an interstellar spacecraft would likely be very different from the presented concept. The spherical shell could be a very valuable demonstrator in the vicinity of the Earth. Once we have convincingly shown that interstellar velocities are realistically within reach, it will likely change the research landscape and boost the innovations in ultra-light materials. Aerographite has such remarkable properties, but it was not developed at all for being a solar sail! There is certainly a large margin for improvement through a dedicated research effort.
A black sail presents interesting challenges when we want to track it from Earth. We have an infrared signature to work with that would be compromised by the absorption of atmospheric water vapor, making space-based observation the key. The sail’s effective temperature will drop as it recedes from the Sun, making the wavelength of its peak emission increase. The authors calculate that the James Webb Space Telescope could track such a sail, and demonstrate this by calculating its temperature in thermal equilibrium with absorbed sunlight.
The result: JWST observations of an aerographite sail of 1 m radius are possible out to 2 AU. A sail 10 m in radius can be observed to 3 AU. But we have other options as we move into the outer Solar System. A swarm configuration of sails as discussed yesterday could, the authors believe, be tracked in deep space by ALMA (the Atacama Large Millimeter/submillimeter Array) at distances of 1000 AU and beyond as the sail’s temperature drops to 10 K.
We’re hoping, of course, for a communicative spacecraft, one relying on ultra lightweight instrumentation. Ideally we would want to implement a laser on-board to remain in contact with Earth. From the paper:
Miniaturization of electronic components has made great progress in the last few decades, but we focus on mass margins above 1 g because we do not expect sub-gram margins to be relevant for the foreseeable future. Commercial lithium-ion batteries weighing a few grams and with power densities > 1 kW kg?1 (Duduta et al. 2018) as well as ultra-high-energy density supercapacitors with power densities of ?32 kW kg?1 (Rani et al. 2019) are already available, allowing energy emission of a gram-sized power source of 32 W in theory.
And again:
A laser sending the proper time of the sail to Earth would allow distance and speed measurements through the relativistic Doppler effect. Measurements of gravitational perturbations (Christian & Loeb 2017; Witten 2020) under consideration of dust and gas drag as well as magnetic forces exerted from the interstellar medium (Hoang & Loeb 2020) could also be used to search for the suspected Planet Nine in the outskirts of the solar system. Its expected orbital semimajor axis is between about 380 AU and 980 AU (Batygin & Brown 2016; Brown & Batygin 2016).
An interesting thought! René Heller mentioned this as well in an email, talking about the prospect of hundreds or thousands of aerographite sails, each with a gram-sized on-board transmitter to allow tracking of distance and speed relative to Earth. A reconstruction of their individual trajectories could be used to look for gravitational perturbations leading to the putative Planet Nine. Remember, this is a low-cost material. The authors estimate that meter-sized aerographite spheres with a thickness in the ?m range could be produced in large numbers for roughly $1000 US. Breakthrough Starshot is using a per-sail cost estimate of $100 US.
Let’s look long-range again and consider an interstellar implication. If aerographite did allow a mission to, say, Proxima Centauri with a travel time of less than 200 years, would there be any way to decelerate it upon arrival? Obviously we could brake against the star’s light, but the problem with Proxima is that its light is relatively weak, and deceleration would be negligible. Heller and Hippke have in the past considered using Centauri A and B as buffers for deceleration, with the residual kinetic energy absorbed by Proxima Centauri itself (see By ‘Photogravitational Assists’ to Proxima b).
But I’m getting way out in front in going this route. What we need now is something we can deploy in the near-term, and here it’s conceivable that aerographite may become valuable even for a laser-beaming project like Breakthrough Starshot. From co-author Guillem Anglada-Escudé (Institut de Ciencies Espacials, Barcelona):
…we are aiming at something we can deploy immediately, at a low budget. As you know, space is very slow in demonstrating technology. But most unknowns can be sorted out if we can make it fly instead of theorising about it for two decades. That’s the spirit. All knowledge on operating sails is of high value anyway. The micro-instrumentation that Starshot needs can be installed, tested and begin producing science with these sails on Solar System exploration for example.
A rousing prospect, that. An early hollow sphere aerographite demonstrator with a diameter in the range of a few meters might be brought into space as a piggyback add-on to an existing interplanetary mission, adding little mass given the lightweight nature of the material. Add to the low cost of aerographite itself the fact that deep space missions, conceivably interstellar ones, can be implemented using solar photons alone, without the need for a massive laser installation and all the issues ground-based laser beaming introduces, and the economic justification for pursuing this research becomes obvious.
Moving a small sample of aerographite with light in the laboratory is the next step in the research, a set of experiments now being devised. Breakthrough Starshot should be keeping an eye on this research.
The paper is Heller, Anglada-Escudé, Hippke & Kervella, “Low-cost precursor of an interstellar mission,” Astronomy & Astrophysics 7 July 2020 (abstract / preprint). The aerographite discovery paper is Mecklenburg et al., “Aerographite: Ultra Lightweight, Flexible Nanowall, Carbon Microtube Material with Outstanding Mechanical Performance,” Advanced Materials Vol. 24, Issue 26 (12 June 2012). Abstract.
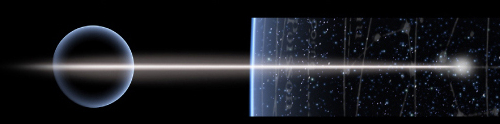
by Paul Gilster | Jul 29, 2020 | Sail Concepts |
Aerographite is an ultra lightweight material made of carbon microtubes, just the sort of thing that seizes the imagination in terms of material for space sails powered by solar photons or laser beam. Such materials are much in my thinking these days and have been for some time, ever since I first read some of Robert Forward’s papers on using laser beaming to boost enormous sails to a substantial fraction of lightspeed. What kind of materials would be used, and how could the mass be kept low enough to allow significant payloads to be deployed?
These days, we think in terms of much smaller sails with miniaturized payloads of the sort advocated by Breakthrough Starshot. But of course advances in sail technology enable a wide range of concepts, and the place to start is with laboratory experiment — this is where we are with aerographite right now — moving into space demonstrators that can be low-cost and near-term. The kinds of missions conceivable with aerographite include fast access to the outer Solar System and, with the help of a close solar pass, interstellar trajectories to nearby stars.
What we are examining in this series of posts is a concept paper that asks for the first time whether aerographite can become a sail material, noting its low cost and our ability to begin testing not just on the ground but in space to find out whether it can carry a payload and survive the stresses of deep space journeys. Lead author René Heller (Max Planck Institute for Solar System Research, Göttingen), with co-authors Guillem Anglada-Escudé (Institut de Ciencies Espacials, Barcelona), Michael Hippke (Sonneberg Observatory, Germany) and Pierre Kervella (Observatoire de Paris), offer a pointer to areas of investigation we will have to address.
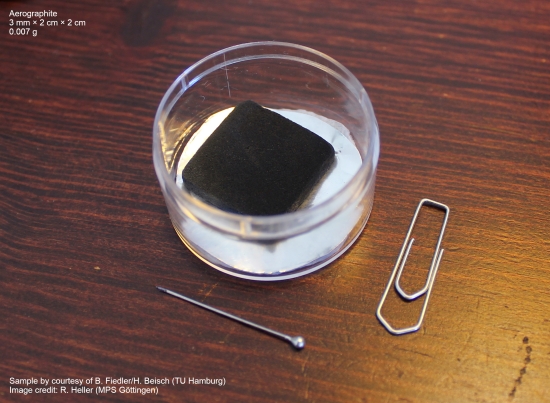
Image: An aerographite sample from the Technical University of Hamburg now under study as its potential as a sail material is examined. Sample courtesy of B. Fiedler/H. Beisch (TU Hamburg). Image by R. Heller (MPS Göttingen).
Exploring Aerographite’s Potential
When the teams at the Technical University of Hamburg and the University of Kiel developed aerographite, they dubbed it “the lightest known material.” It is a synthetic foam connected by carbon microtubes with a density of 180 g/m3. This intriguing material has useful properties indeed, though some of these, as we’re about to see, are problematic. For now, let’s note that aerographite is ultra-black at the 1 mm scale considered in the Hamburg team’s paper (citation in yesterday’s post), though as Guillem Anglada-Escudé explained, its opacity is unclear as we go below that scale, a matter that will have to be addressed.
Aerographite’s opacity is important because we are talking about a sail that functions not through reflectivity but absorptivity. The sail concepts we’ve bandied about in these pages have for the most part revolved around reflection, so let’s pause on this. I was unsatisfied with my own description of an absorptive sail in a rough draft of this post, so I asked René Heller for his own take. An opaque sail, he replied, absorbs any light that impinges upon it:
Think of a shower of photons that bumps into an aerographite sample. The sample is almost entirely black, and so most of the photons will not be reflected but they will transfer their kinetic energy (and their momentum) to the sample. As a consequence, the sample will be heated and “pushed” into the same direction as the incoming photon shower was moving before it got absorbed. This is due to conservation of momentum. Now if the sample were reflective then the incoming photons would bounce back from the sample in some angle. If the sample were 100% reflective and if it had a perfectly flat surface, and if the photons were coming in in a perpendicular angle with respect to the surface, then the sample would receive twice the amount of the momentum that it received in comparison to the absorptive case described above. In other words, reflection is twice as effective as absorption.
And yet absorption in the right material can be enabling. While we begin deeper investigations into aerographite’s properties, it’s worth noting that the 1 mm shell thickness in the putative sail explored in these calculations is already useful for some mission concepts. Solar photons alone can push an aerographite sail of this thickness beyond Solar System escape velocity.
If laboratory work confirms that the shell can be reduced below 1 mm while remaining opaque, we can start talking about on-board technology like scientific instruments and the needed communications devices. We can also start talking about much faster transit times. As mentioned yesterday, Heller et al. find the orbit of Mars reachable within 60 days assuming an aerographite shell of 0.5 mm. That gets you to Pluto in 4.3 years, nicely halving the New Horizons travel time. As you can see, we are getting into interstellar precursor range.
This is done through solar photons alone, without the considerable overhead of the massive laser array assumed by Breakthrough Starshot, with obvious (and enormous) cost savings. Starshot wins on speed, aiming for 20 percent of lightspeed and thus a transit time to Proxima Centauri of about 20 years. But put a 1 ?m thin aerographite hollow sphere at 0.04 AU (this is Parker Solar Probe territory) for a close solar pass and you achieve 6900 km/second, which gets you to Proxima Centauri in 185 years, well below the 1,000 year threshold thus far determined for a graphene sail.
How to make a sail out of this material? Anglada-Escudé explained in an email that aerographite:
…has structural integrity (it keeps its shape and recovers over deformation), and it is “relatively” simple to make. Think of it as a foam. You make a template of porous ZnO [zinc oxide], carbon fills in the gaps through a deposition process forming carbon fibres/tubes/sheets, etc. You remove the template material, and you have a block of very light aerographite foam with the shape of the original ZnO template.
The authors discuss hollow spheres as the sail’s shape, but a range of possibilities exist. The spherical shape was chosen for the paper because it provides the simplest solution at this stage of the evaluation of aerographite, but a cone is likewise feasible, and would likely provide stability — I think of this in terms of Jim and Greg Benford’s work on ‘beamriding’ sails, obviously an issue with Breakthrough Starshot.
The today typical ‘flat’ sail is likewise a possibility. The authors have discussed the matter with the Hamburg group behind aerographite, with the latter seeing no problem in manufacturing the material in arbitrary shapes and sizes. Adds Anglada-Escudé:
A spherical shell with a wall of 1 mm is already unbound to the Solar System, but if we could work with a more classic flat (or quasi flat) sail in ‘parachute’ configuration, we could work with up to 4 mm in thickness (which is pretty macroscopic) and add payload easily. I would think that the idea is to start the conversation and let the community evolve it to the most useful application. This is now mission planning, not speculative design! Deploying it would be so cheap… it is almost painful to think about why we have not done it yet.
Or we can get more exotic still, as lead author Heller told me, envisioning separate sail components connected by carbon nanotubes:
The shell (or hollow sphere) design that we focus on is just better than a solid sphere or a cube but not necessarily the optimal shape. I personally think a web of dozens or hundreds of cm-sized cones or parachutes, all of which automatically orient themselves to the solar radiation individually will be a more practical application if it really comes to moving payload through the solar system. Such a web of aerographite parachutes would be more resilient to failure (of one or a few parts) and it would allow larger mass margins for the payload. I wouldn’t pay too much attention to single-shell concepts for real applications, although hollow spheres make the math very simple, which is why we used them for this introductory paper. A web will be better for “large” (gram to kilogram) payloads.
Directions for Research
Aerographite gives the appearance of an ideal sail material if it can be used to carry a payload. The authors produce benchmark scenarios showing that the weight of the payload is 1000 times the mass of the transport system, quite a contrast with chemical rockets — think New Horizons and its Atlas V — where the transport system is 1000 times heavier than the payload.
The authors calculate payload mass in terms of shell thickness, using benchmarks of 1 ?m and 100 ?m, with this result:
…a 1 m radius hollow aerographite sphere with a shell thickness of 1 ?m (100 ?m) would weigh 2.3 mg (230 mg) and have a margin of 2.4 g (2.2 g) to get interstellar. Upon release to the solar radiation in interplanetary space at 1 AU from the Sun, a payload mass of 1 g would yield a terminal speed of 51 km s?1 (41 km s?1), which is 3 times (2.4 times) the terminal speed of Voyager 1. A travel to the orbit of Pluto would take 3.9 yr (4.7 yr). An increase of the sail radius to 5 m would allow payload masses of 10 g to reach the orbit of Pluto in almost half the time.
These are tiny payloads, which is why the authors consider the aerographite sail in terms of scalability. The notion of a swarm of aerographite spheres connected by carbon nanofibers — “the additional weight of which would be small compared to the mass of the aerographite shell” — implies a combined thrust that would allow larger payloads on the one hand, or faster travel time for a given payload. Payloads could thus be brought into the kg domain if sail sizes in the 100 meter range can be achieved.
One advantage of this concept is that it would aid in tracking the spacecraft, which is itself an issue we need to address. But so is the matter of how a payload is positioned, either attached to the sail itself or, depending on sail shape and configuration, drawn behind it in parachute fashion. This is a problem faced by Breakthrough Starshot as well as it seeks the right sail material and optimum configuration for ‘beam-riding’ with its proposed laser array. The authors do not address the question of payload configuration and attachment in this paper.
Nor are we through with significant issues. How would the aerographite sail behave in space, given its tendency to accumulate electrical charge? Navigation issues immediately arise that we need to talk about tomorrow, and we also have issues regarding aerographite’s tensile strength.
Let’s also talk in the next post about the kind of missions we might want to deploy, assuming a successful research project produces flight-testable sails. Interesting options open up long before we start talking about true interstellar flight, perhaps piggybacking on other missions as we learn both about the material in space as well as the formidable issues of tracking an utterly black sail. More on all this next time.
The paper is Heller, Anglada-Escudé, Hippke & Kervella, “Low-cost precursor of an interstellar mission,” Astronomy & Astrophysics 7 July 2020 (abstract / preprint).
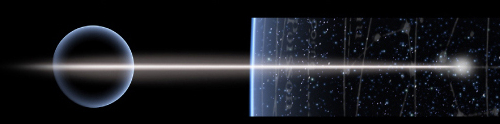
by Paul Gilster | Jul 27, 2020 | Sail Concepts |
Invented at the Technical University of Hamburg and developed with the aid of researchers at the University of Kiel, a new material called aerographite offers striking prospects for solar sail missions within the Solar System as well as interstellar precursor implications. Judging from the calculations in a just published paper in Astronomy & Astrophysics, aerographite conceivably enables a mission to Proxima Centauri with a flight time of less than two centuries. We are not talking about laser-driven missions here, but rather meter-scale craft that would be pushed to interstellar velocities by solar radiation; i.e., true solar sails.
But let’s focus near-term before going interstellar. I’ve been talking to René Heller (Max Planck Institute for Solar System Research, Göttingen) about the paper, along with co-authors Guillem Anglada-Escudé (Institut de Ciencies Espacials, Barcelona), Michael Hippke (Sonneberg Observatory, Germany) and Pierre Kervella (Observatoire de Paris). Just what are the prospects for aerographite, and what are its problems? The authors stress that aerographite for space applications implies a development path through laboratory work and near-term experimentation in space. “Before we run, we need to walk,” as Anglada-Escudé told me in an email that summarized how the idea grew.
Anglada-Escudé and Heller had been studying the conditions that would allow a sail pushed by solar radiation, as opposed to laser beaming, to leave the Solar System, developing calculations for the kind of material needed, and initially envisioning a sail made of graphene (about which more in a moment). As Hippke and Kervella joined the discussion, the connection with aerographite was made and the previous computations recalculated. Says Heller:
“Aerographite is both ultralight and opaque (= black) so that it effectively absorbs photons and overcomes the gravitational attraction from the Sun under certain circumstances. We find that a hollow sphere (or shell) with a diameter of a few meters and a shell thickness less than 1 mm would become unbound from the solar system if it were submitted to sunlight in interplanetary space. It could be brought into space as a piggyback mission to any interplanetary mission without adding significant amounts of mass to the payload (because aerographite is so ridiculously lightweight)”.
Adds Anglada-Escudé, on how a material might be ‘unbound’ from the Solar System:
It is known that small dust (mostly SiO2 [silicon dioxide] balls, which are quite dense) in the solar system are blown away at some 100 nanometer sizes, so any material lighter than that should work. Also, one does not need to be reflective. Absorbance is also OK (only a factor of 2 worse than a fully reflective material).
The reference to Starshot is useful because it sets up some technology comparisons I want to make, while also driving home the point that as a near-term goal, something as relatively “local” as a demonstrator released from the International Space Station could move the ball forward. So what I want to do in the course of the next several posts is to examine the Astronomy & Astrophysics paper and consider the uses of aerographite in a variety of mission concepts as we begin to explore how such a sail could be constructed and flown.
But we also need some context, and a nod to a slightly earlier and itself promising sail material gets the story in motion.
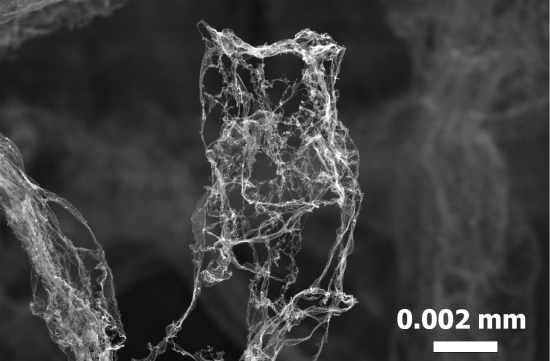
Image; This graphic shows a detail of the world’s lightest material: aerographite. Open carbon tubes form a fine mesh and offer a low density of 0.2 milligram per cubic centimetre. The picture was taken with a scanning electron microscope (SEM). Credit: TUHH.
Sails for Deep Space Missions
Sails have been considered for long-duration, conceivably interstellar missions since the days of Robert Forward, and Centauri Dreams readers will also be aware of such seminal works as Greg Matloff’s 1981 paper “Solar Sail Starships—The Clipper Ships of the Galaxy,” which ran in the Journal of the British Interplanetary Society. What we are after is a thin sail that is temperature tolerant and rugged enough to endure its passage through the interstellar medium. Such a sail is usually assumed to be highly reflective, though aerographite will put a new spin on this. A ‘sundiver’ maneuver, taking a shielded sail close to the Sun for deployment at perihelion, was seen as offering travel time to the Centauri stars of perhaps a thousand years. And travel times like that seemed the best we could do with solar sails.
Laser-beaming could conceivably change the equation, and the Breakthrough Starshot effort revolves around a massive, ground-based laser array that would drive small sails up to 20 percent of the speed of light for fast passage to Proxima Centauri or other stars. In any case, the question of materials figures prominently in sail literature. The most studied material to date has been beryllium, but in 2012 Greg Matloff revisited sails with graphene in mind. He described it as “a mono-molecular lattice of carbon atoms” and noted that materials experts and condensed matter physicists had graphene under intense investigation. Matloff saw prospects for graphene in terms of thin-film probes and much larger manned starships:
In its application to interstellar solar sailing, it seems that graphene can exceed the performance of beryllium with less extreme perihelion requirements, peak temperatures and maximum accelerations. Thousand-year transits to Alpha Centauri do not seem out of the question for probes and generation ships using this mode of acceleration and deceleration.
Matloff also noted the problems posed by graphene, pointing to the difficulty of large-scale preparation at high-purity levels and questions involving its performance during a close solar pass, a maneuver that colleague Roman Kezerashvili had analyzed for beryllium sails several years earlier. The question facing Heller, Anglada-Escudé, Hippke and Kervella was whether the newly discovered aerographite could significantly upgrade the performance of a graphene sail, allowing us to reduce travel times to something below that 1,000 year threshold.
Aerographite offered several clear advantages along with some properties that would need to be analyzed and accounted for. It is true that graphene’s extremely low mass per cross section ratio can theoretically enable high velocities. But graphene turns out to be all but transparent, with a reflectivity close to zero. And now we run into difficulties with creating a graphene sail that I want to illustrate by quoting the Heller et al. paper. In the passage that follows, sigma (?) stands for the mass per cross section ratio, which for graphene is 7.6 × 10?7 kg m?2:
The absorptive and reflective properties of graphene can be greatly enhanced by doping graphene monolayers with alkali metals (Jung et al. 2011) or by sandwiching them between substrates (Yan et al. 2012). But this comes at the price of greatly increasing ?. The limited structural integrity of a graphene monolayer requires additional material thereby further increasing ? and complicating the experimental realization. All of this ultimately ruins the beautiful theory of a pure graphene sail.
To be sure, Matloff and others working on the graphene sail concept are well aware of these issues and various papers have investigated the prospects for surmounting them. But the prospect of an aerographite sail, a material with its own strengths and question marks, gives us a new entrant in the sail arena, now with initial calculations provided by Heller and team. Specifically, what the Astronomy & Astrophysics paper does is to examine a hollow sphere at meter-scale, one made out of aerographite, that can be launched into interplanetary space by conventional rocket and released, allowing solar photon pressure to go to work.
Missions within the Solar System assuming such a sphere with a shell thickness of roughly 0.5 mm could, according to these calculations, reach the orbit of Mars within 60 days, arriving at Pluto’s orbit in 4.3 years. If the material proved capable of withstanding a close solar pass, there are mission prospects for Proxima Centauri in the range of 185 years of travel time.
But a Proxima mission gets way ahead of the game. We need to look at aerographite in terms of what we can learn about it in the near-term, with missions within the Solar System of various complexity part of the learning curve. In the next post, I want to go into how an early aerographite sail could be made, and how deep space sails might be configured, and we’ll consider whether in the near-term, solar as opposed to laser sailing may be our path toward true interstellar precursors. And either tomorrow or in a third post, we need to examine how to overcome some problems raised by this material in terms of both navigation and monitoring / communications.
The paper is Heller, Anglada-Escudé, Hippke & Kervella, “Low-cost precursor of an interstellar mission,” Astronomy & Astrophysics 7 July 2020 (abstract / preprint). The discovery paper for aerographite is Mecklenburg et al., “Aerographite: Ultra Lightweight, Flexible Nanowall, Carbon Microtube Material with Outstanding Mechanical Performance,” Advanced Materials Vol. 24, Issue 26 (12 June 2012). Abstract. Matloff’s paper on graphene is “Graphene: The Ultimate Interstellar Solar Sail Material?” JBIS 65, pp. 378-381 (2012) (full text).
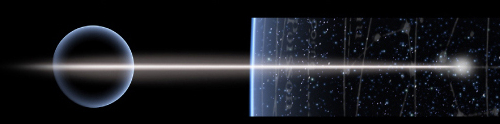